Developments in Rice Research: Visions and Pragmatism
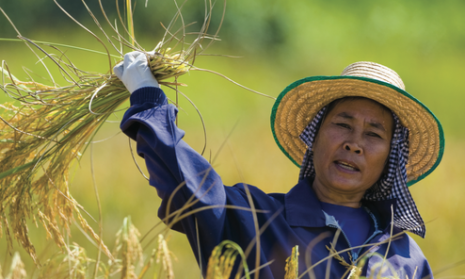
Summary
Rice research concerns the development of new products, concepts and technologies to improve the yield and quali- ty of rice in ways that do not damage the environment. Over the next 40 years, the predicted 2.2 billion (32%) increase in the number of human beings on the planet threatens the ability of agricultural technologies to produce suf- ficient food to meet the demand.
Billions of people eat rice every day and it is the main source of energy and protein for hundreds of millions of poor people. Almost all of the world’s production of rice, 690 million tonnes, is consumed by humans and over the past half century, there has been a linear relationship between population in Asia and rice production. The population in Asia, where sixty percent of the world’s population live, will increase by about a billion over the next half century and rice production may have to double to keep pace, whilst overcoming some of the nega- tive effects of climate change.
Additional research to protect yields from high temperature damage will require further research on heat tolerance and on breeding rice cultivars that flower in cooler parts of the day. Unfortunately, the elite rice cultivars of the Green Revolution have approached a yield barrier and the increase in yield/ha is slowing.
There is rising competition for water between agriculture, human consumption and industry. Given the abundant use of water for most rice production, availability could be a significant future constraint. The yield barrier would be broken by changing the photosynthesis of rice from the C3 to the C4 metabolic pathway. Such a change would be accompanied by a near doubling of water-use efficiency and an improvement in nitrogen-use efficiency.
We argue that there is an urgent need for transformative research aimed at breaking existing yield barriers and improving nutritional quality of the grain. We describe the objectives of an international effort aimed at producing C4 rice. There is an urgent need for funding the high-risk, high-return research that will enable us to dissipate the problems facing human- ity before those problems engulf us.
Keywords: Rice, photosynthesis, molecular biology, water, pests, weeds, climate change, biofortifica- tion, funding, C4 rice
Introduction
Today, 75% of the world’s 6.8 billion people live in the developing world, where most of the world’s poverty is concentrated. A billion people live on about a dollar a day and spend half their income on food. Some 854 million people are hungry and each day about 25 000 people die from hunger- related causes (Sheehy and Mitchell 2011). Rice, wheat, maize, millet and sorghum provide 70% of the food energy of the world.
About half the world's population has rice as the staple cereal, and almost all of the world production, 690 million tonnes in 2010, is consumed directly as food , particularly in Asia, where 90% of rice production is grown and eaten (Rice Almanac 2002). Clearly rice has a key role in global food security and in efforts to reduce hunger and poverty. Rice production is supported by research around the world, much of it now coordinated in the Global Rice Science Partnership (GRiSP 2010). Rice research contributes to poverty-alleviation through several direct and indirect mechanisms (Dawe 2000).
In his acceptance speech for the Nobel Peace Prize in 1970, Norman Borlaug warned that if the frightening power of human reproduction was not curbed, the success of the Green Revolution would be ephemeral. Since then, world population has already increased by 75% and is continuing to rise. In the 21st century the population of Asia will rise by about 27% to 5.2 billion and that of Africa will almost double to nearly 2 billion.
At the same time the urban fraction of the population is predicted to reach 70% by 2050. Each year, a city of a million people consumes about 0.75 million tons of food and 117 million tons of water (Stanners and Bourdeau 1995). In addition, about 1.5 billion tons of water (rainfall or irrigation) are also used in producing the food for that city. By 2050, the urban population will require about 4.7 billion tons of food annually and the rural population, upon whom the urban population depends for its food, will require about 2.0 billion tons annually.
How to feed the rapidly growing population of the world in a sustainable manner would be a daunting challenge for world agriculture even in the absence of climate change. Global food security and the need to eliminate hunger and poverty have become topics of general conversation and the subject of several recent reports (Royal Society 2009; Godfray et al. 2010; Foresight 2011).
The combination of increasing population, rapid economic development in Asia and South America, climate change, and neglect of funding for agricultural research threatens food security and therefore the stability of society. The question arises: what proportion of the world's population has to be hungry before civilization as we know it ceases? We do not wish to find out the answer to this question; instead efforts must be concentrated on increasing food production and improving distribution to meet the demand, doing this sustainablywhilst achieving resilience in response to climate change.
The situation can be summarised in a sentence. We must produce more rice, of higher quality, from less land, using less water, less labour, less fertiliser, less pesticide, and with a smaller carbon footprint during an era of climate change.
It is worth noting that this eclectic set of criteria includes some of the classical economic factors of production (land, labour, capital) and some of the biological resources needed by plants (solar radiation, carbon dioxide, water, mineral nutrients). However, business as usual will not be good enough in the pursuit of increased rice production.
In the early nineties it was recognised that elite rice cultivars had a yield barrier (Kropff et al. 1994); this is the reason that the gains of the Green Revolution are largely exhausted (Fig. 1; Sheehy 2001a; Sheehy and Mitchell 2011). Between 1961 and 2001 the production of rice kept pace with Asia's population, but to keep up in the face of climate change over the next 40 years will require breaking this yield barrier.
An option is to install a C4 photosynthetic system in rice (Mitchell and Sheehy 2006). In this paper, we urge the beginning of a new era of high- risk, high-return, transformative research, a large part of which should be focused on a substantial increase in yield. We concentrate on biological research topics, selected because they derive from systems analysis, or rigorous quantitative assessment, and are intended to be transformative rather than simply incremental.
Glossary
Activation tagging: Insertion of activation vectors carrying strong promoters causing mutagenesis; designed to generate novel phenotypes.
Aerobic rice: Rice varieties that would normally be grown flooded shallowly, instead being grown in soil without flooding, i.e. with irrigation merely to maintain a moist but aerobic soil.Alternate wetting and drying: A technique to minimize use of irrigation water by flooding the rice field followed by drainage until the soil is at field capacity and then reflooding. Backcrossing: In genetics, crossing the hybrid between two varieties with one of the parent varieties, and repeating this to obtain all the characteristics of the backcross parent plus one or more characteristics from the other parent. Biofortification: Production of staple food crops that contain a selected micronutrient in concentrations higher than normal.
Bioinformatics: Use of databases and associated tools to store, manipulate and analyse biological data, in particular sequences of DNA and genetic information.
Biological nitrogen fixation: Capture of gaseous nitrogen from the atmosphere into biological compounds; carried out by certain bacteria either living freely in soil or in symbiotic association with a green plant.
Bund: A low earth bank to retain irrigation water on a field; such a field or section of a field is bunded.
Bundle sheath cell: Cells forming a sheath around the vascular bundles (veins) of vascular plants. In C4 plants these cells become highly specialized for part of C4 photosynthesis.
C3 (photosynthesis, plant): The basic mechanism of photosynthesis where the first detectable compound in which CO2 is fixed has three carbon atoms.
C4 (photosynthesis, plant): The kind of photosynthesis where the first detectable compound in which CO2 is fixed has four carbon atoms; the CO2 is released and refixed by the C3 process in a different cell.
Compensation point (for CO2): The concentration of CO2 at which photosynthesis balances respiration by a leaf, in defined conditions.
Cultivar: A named and registered variety of cultivated plant, the product of plant breeding.
Dry deposition: Gases or particles in the atmosphere arriving at a surface without involvement of water; opposite of wet deposition where rain or snow is involved.
Evapotranspiration: Loss of water to the atmosphere from vegetated ground from a combination of evaporation from the soil and transpiration through the plants.
Field capacity: The water content of a soil when water stops draining from it under the influence of gravity alone. Germplasm: Generic term for any source of genetic variation for use in plant breeding, whether existing varieties, landraces or related species.
Greenhouse gas: Any gas in the atmosphere that absorbs infra-red radiation emitted by the surface of the earth and thereby keeps the earth warmer than it would be if the radiation escaped directly to space. This includes water vapour, carbon dioxide, methane, nitrous oxide and several other compounds. Given that water vapour is universally present it is carbon dioxide, methane and nitrous oxide that attract most attention from the point of view of climate change because their concentrations have been measurably changed during the last 250 years since the start of the Industrial Revolution. Harvest index: The harvested portion of a crop as a proportion of all the crop above ground, each measured as dry weight.
Husk leaves (of maize): The leaves that surround the cob, as opposed to the foliar leaves borne on the stem.
Indica: The tropical subspecies of rice, as opposed to the temperate one (japonica).
Kranz anatomy: The characteristic pattern seen in transverse section of the leaf of a C4 plant where there is a dark green halo around each vein, standing out from the paler green colour of the rest of the leaf; from the German word (curiously retaining its Germanic capital after nearly a century of usage) for a wreath.
El Niño and La Niña: are the names given to periodic climatic patterns that occur across the tropical Pacific Ocean; El Niño is associated with very low rainfall and high radiation conditions in the Philippines, La Niña is associated with high rainfall and low radiation. Landrace: Variety of a crop plant that has not been consciously selected or bred but arisen from continuous selection and use by farmers in a particular place.
Lodging: The toppling over of a cereal plant to a near horizontal position by heavy rain or wind or both.
Logistic growth: A pattern of growth which is slow at first, then rapidly increases and then levels off to a plateau; that can be described by a logistic equation.
Marker-assisted (backcrossing, breeding): The ability to select plants with the desired characteristic before that characteristic becomes apparent by using biotechnological techniques to identify a piece of DNA, the marker, that is closely associated with the gene for the characteristic.
Mesophyll cell: The cells in the middle of a leaf, containing chloroplasts. In C3 plants all photosynthesis is carried out in these cells. In C4 plants only the first part of C4 photosynthesis occurs in the mesophyll cells.
Micronutrient: An essential nutrient required in small amounts in the diet, of the order of micrograms or milligrams daily, as opposed to protein, carbohydrates and fats required in bulk.
Mid-season drainage: The practice in managing irrigated rice of draining the field completely in the middle of the growth of the crop and then reflooding.
Nitrogen use efficiency: A measure, variously defined, of the proportion of nitrogen in a crop relative to what was supplied as fertilizer or what was derived from the soil or both.
Panicle: The much branched inflorescence of the rice plant (and many other grasses) with grains borne on the tips of the branches.
Phenology: The study of the timing of biological events such as flowering, in relation to environmental factors. Promoter: A length of DNA that switches on a gene next to it.
Quantitative trait locus: The location of the DNA (locus) that contributes genetically to a characteristic (trait) that is variable in amount (quantitative) instead of qualitative; loosely, one of the genes for the characteristic.
Radiation use efficiency: The amount of dry weight of a crop (usually, but could be the energy content of the dry weight for a true efficiency) that is produced from unit amount of radiation on the crop. Numerical values must have precise definitions of the parts of the crop measured, the part of solar radiation considered, and whether the radiation was absorbed or intercepted by the crop.
Tiller: A branch of a grass plant, hence of cereal crops, arising from a bud near the ground.
Transcriptome: is the set of all RNA molecules produced in one, or a population of cells.
Transgenic: A plant containing a gene from a different species moved into it by genetic engineering.
Transpiration: Loss of water from the leaves of a plant as a continuous flow from the roots.
Up-regulate: Adjustment of metabolism upwards or increased in some way in response to change in an environmental factor.
Variety: Loosely, a cultivar; technically in botanical nomenclature a named variant of a species of plant which occurs in the wild and breeds true.
Vein (in leaf): The feature on a leaf visible with the naked eye that marks the presence of a vascular bundle, the transport system of the plant for water and sap.
Water use efficiency: A measure, variously defined, of the proportion of water used by a crop relative to what arrived as rainfall or was supplied as irrigation or both. The numerical value is strongly dependent on the scale of measurement and time period, whether for a leaf, a plant, a crop or field, or a catchment; and whether instantaneous, for 24 hours, for crop duration or for a year.
Yield potential: The yield of a variety in an optimal physical environment (solar radiation, temperature, water, mineral nutrients) and with complete protection from weeds, pests and diseases.
Abbreviations
BS bundle sheath, CGIAR Consultative Group on International Agricultural Research, DW dry weight, GHG greenhouse gas, GRiSP Global Rice Science Partnership, HI harvest index, IPM integrated pest management, IRRI International Rice Research Institute, M mesophyll cell in C4 plant, MJ megajoules, i.e. 106 joules, NOAA – ESRL National Oceanic and Atmospheric Administration – Earth System Research Laboratory, Rubisco ribulose 1,5-bisphosphate carboxylase–oxygenase, RUE radiation use efficiency.
Rice ecosystems
A little over a third of the world’s land is suitable for agriculture; the rest is ice, desert, forest or mountains unsuitable for farming. In 1950, the world’s population was about 2.3 billion and there were about 2 ha of farmland available to meet the food requirements of each person. By 2050 the available farmland will have fallen to less than 0.6 ha/person, assuming forests and wetlands remain free of agriculture.
Rice is grown on every inhabited continent, in a wide range of climates, in four ecosystems characterised by the presence or absence of surface water (from flooded to dry land) for all or parts of its growing season. The contributions of the four ecosystems to the world totals are shown in Fig. 2.
The area available for rice cultivation is likely to decrease as land is taken out of cultivation for industry and housing as urbanisation proceeds. One consequence of the very unequal distribution of rice production among the ecosystems is the different emphasis of research in each ecosystem for either food security or for alleviating hunger.
From the point of view of food secu- rity, the bulk of world rice production comes from the irrigated system and must continue to do so. Rainfed low- land rice in favourable locations and Research here concentrates on improving productivity from larger farms with good access to inputs, information and markets.
In contrast, rice-eating communities that tend to grow rice in the upland, flood-prone or in unfavourable rainfed lowland ecosystems are vulnerable to hunger. Supporting smallholder agriculture is vital for these communities and is the focus of research for direct application in these situations, and also the object of much attention from charitable organisations.
Research from bottom- upwards or farmer-led initiatives have a role here because small interventions carefully identified can produce critical improvements in crop survival, reliabil- ity and yield. Local solutions and low- input systems may be entirely appro- priate in these cases.
Constraints Water
Only 1% of water on the planet is fresh and there is rising competition between agriculture, human consump- tion and industry. Given the abundant use of water for most rice production, water availability could be a significant constraint. Bouman et al. (2007) review the use of water for rice, from field to catchment scale, present and future.
Water vapour moves from inside leaves to the atmosphere (transpira- tion) while carbon dioxide takes the reverse path (photosynthesis). Since the gaseous diffusion pathways are the same, there is a near-linear relationship between transpiration and photosyn- thesis (deWit 1958). Evaporation from the soil, or water surface, is described mathematically in terms of vapour pressure deficit, wind speed and radia- tion (Penman 1948); these factors also affect transpiration.
As the crop canopy develops, the balance between evaporation and transpiration changes, but,not surprisingly, there are close empirical relationships between yield of biomass and evapotranspiration (Tanner and Sinclair 1983; deWit 1958; Connor et al. 1985). Improvements in the productivity of current terrestrial crops must be accompanied by proportionate increases in the use of water.
Chapagain and Hoekstra (2011) esti- mate the global requirement of water for rice to be 784 km3 year-1 of which 44% is from direct rainfall. In Asia, irri- gated rice accounts for 50% of the water diverted for irrigation (Tabbal et al. 2002). Future demands for irriga- tion water for rice will compete with an increased use of water by industry and urban development.
Despite the components of the water balance being well understood, it is not easy to calculate accurately the amount of water required for global rice produc- tion because of variability in soils, weather and climates. Irrigated rice requires about 150–250 mm water for land preparation, 50 mm for seedling growth in the nursery, and 5–15 mm day-1 for evapotranspiration from transplanting to harvest (Guerra et al. 1998; De Datta 1981).
In addition, there are losses from percolation through the puddled soil, varying between 1 and 30 mm day-1 depend- ing on soil type (Bouman and Tuong 2001). A certain minimum percolation is required to ensure that toxins do not accumulate in the rooting zone, but the quantity is neither well defined nor is it easily controlled (Aimrun et al. 2010; De Datta 1981). It can be seen that there is great variation in the esti- mates of the components of water use for rice (Shashidhar 2007; Zwart and Bastiaansen 2004).
It is clear that more site-specific reliable measurements of all the components of the water bal- ance are required before estimates of future demands for the irrigated sys- tem can be made with confidence.
Many areas of the world grow rice without irrigation in bunded fields that capture and retain rainwater (rainfed lowland rice), or in unbunded fields (upland rice). These regions often receive near adequate rainfall for max- imising rice production during the growing season (Thiyagarajan 2001). Indeed, in many countries the water used for rice farming comes mainly from rainfall in the wet season, so sur- prisingly, the contribution of rice to water scarcity is relatively small (Chapagain and Hoekstra 2011).
Major problems in rainfed lowland and upland systems are usually unpre- dictable rainfall, infertile soils and weeds. Even in bunded fields, and with uniformly distributed rainfall, water shortage can occur and reduce yields significantly. Under such circum- stances it is the magnitude of the decrease in water use efficiency which should become the focus of research attention.
Yields are severely restricted when soils fall below field capacity or when there is no standing water dur- ing reproductive growth, a growth phase especially sensitive to water shortage. It is unlikely that the major advances in yield production required in the next 50 years will come from rainfed environments unless a large improvement in transpirational water- use efficiency can be made. Sheehy and Michell (2006) showed that a change from C3 to C4 rice would increase transpirational water-use effi- ciency by 89%.
The near doubling of the transpira- tional water-use efficiency of rice asso- ciated with a change from the C3 to C4 pathway would be advantageous in both the irrigated and non-irrigated systems. Possible improvements in water use efficiency resulting from increases in crop photosynthesis, caused by increases in atmospheric CO2 concentrations, are likely to be limited by increases in evapotranspira- tion driven by increased atmospheric temperatures (Allen et al. 2003).
Rice does not use more water in transpira- tion than comparable crops and it can be grown with much less water (rain- fall and irrigation) as aerobic rice or with alternate wetting and drying (Bouman et al. 2007). These tech- niques can save 15–45% of water compared with normal flooded growth but yields are reduced by about 20%.
The management of water is likely to remain a complex issue during the next 50 years and ways of raising water-use efficiency at all scales in the farming system will remain important (Bouman et al. 2007). For irrigated rice this will require better irrigation engineering and better use of water at the farm to catchment scales, possibly by assigning a cost to water, thus helping farmers make better decisions about water use, and ensuring high rates of reuse from field to field.
Deep water and submergence
Although rice roots are adapted to anaerobic soils, the above-ground parts cannot tolerate submergence for long, so that too much water on the field can be as damaging as too little. Recent research has been remarkably successful in tackling this problem. Four to nine million hectares are culti- vated with deepwater and floating rice types that are highly elongated plants which thrive in paddies with water up to 4 m deep (Catling 1992); they yield about 1.5–3.5 t ha-1 grain.
Pronounced elongation growth (up to 25 cm day-1) in response to submer- gence maintains sufficient aerial tissue above the water for efficient photosyn- thesis and oxygen exchange with sub- merged organs (Bailey-Serres and Voesenek 2008). The rapid elonga- tion response of deepwater varieties has been shown to depend on two genes named SNORKEL1 and SNORKEL2, that are absent from non- deepwater rice.
About 20 million ha of rainfed low- land rice are adversely affected by monsoon flash floods each year which cause the plants to be totally sub- merged. When partially or completely submerged, most rice varieties display a moderate capacity to elongate leaves and the portion of the stems that are trapped underwater. This elongation growth leads to a spindly plant that easily lodges when floodwaters recede.
If the flood is deep, underwater elon- gation growth can exhaust energy reserves, causing death within a mat- ter of days. The development of high- yielding submergence-tolerant lines largely spans the 50 years of research at the International Rice Research Institute (1960–2010).
Landraces with unusual flooding and submergence tolerance but low yields were first reported in the early 1950s and systematically screened in the 1970s (Vergara and Mazaredo 1975; Mackill et al. 1996). Until the develop- ment of molecular tools in the mid- 1990s, the genetic control of submer- gence tolerance remained unclear. The fine mapping of SUBMERGENCE 1 (SUB1), a robust quantitative trait locus in a submergence- tolerant lan- drace from India, enabled marker- assisted breeding of high-yielding rice capable of enduring transient com- plete submergence (Mackill et al. 1993; Mishra et al. 1996). This enabled its transfer by marker-assisted backcrossing into cultivars preferred by farmers (Xu et al. 2004; Mackill 2006).
The SNORKEL and SUB1 genes are very similar in sequence (Hattori et al. 2009), but SUB1 inhibits elongation of leaves and internodes when induced during submergence. This enables sur- vival by reducing carbohydrate con- sumption, thus allowing the plants to recover from submergence (Fukao and Bailey-Serres 2008a). Ideally, rice vari- eties with tolerance during the entire life cycle should be developed, because flooding can cause damage at all growth stages. However, there are no known varieties with tolerance to submergence at flowering or later. In flood-prone tidal areas, farmers would benefit from rice that is tolerant of saline floodwaters.
Pests, diseases and weeds
Integrated pest management (IPM) in rice can be achieved by using in com- bination cultural practices including varietal resistance, conservation of nat- ural predators and parasites of pest species, and pesticides only when eco- logical and economic considerations indicate their value <http://www.knowledgebank.irri.org/i pm, 11th August 2011>. Biological pest control is a population-levelling process in which one species’ popula- tion lowers the numbers of another species by mechanisms such as preda- tion, parasitism, pathogenicity or com- petition.
Biological control has proved relatively successful and safe. It can be an economical and environmentally benign solution to severe pest prob- lems. If the value of the yield loss is less than the cost of the chemical con- trol methods then biological control should be the management system of choice. The economics of yield losses in relation to the costs of pesticides and their potential damage to human health will always require scrutiny and every new rice cv needs to be thor- oughly tested for susceptibility to pests and diseases.
The large germplasm collections maintained at IRRI have been screened to identify resistance genes and these are being incorporat- ed in most breeding programmes and many modern varieties have multiple resistance. It is certain that as new higher yielding cultivars are bred, issues surrounding the appropriate way of keeping yield losses to pests and diseases at a minimum will contin- ue to be an essential part of the devel- opment process (Rubia and Penning 1990; Heong and Hardy 2008; Heong et al. 1998).
Successful weed management aims to minimise the impact of weeds in the short term and simultaneously to ensure that yield losses will not increase in the long term as a result of practices that are implemented.
Currently, water management plays an important role in weed control in rice so shortage of water leads to weedy and low-yielding crops or to use of other methods of weed control. Weed rice (types of rice that have evolved from varieties grown in much earlier times) pose two problems. First, because many of their characteristics are so close to rice varieties being cul- tivated now, they are difficult to eliminate by cultural methods or by herbi- cides.
Secondly, they could conceiv- ably be recipients through gene flow for characteristics bred into modern cultivars if these convey an advantage to weed rice. Tolerance to herbicides derived from genetically engineered rice varieties is a commonly expressed concern. Molecular methods are required to identify the weed rice types and to assist in the ecological studies of gene flow.
Relatively little is known about pat- terns of phenological development in weeds of rice crops in relation to ele- ments of climate change such as tem- perature and CO2 concentration (Rodenberg et al. 2011) . Flowering can be faster, slower, or unchanged at elevated CO2 concentrations depend- ing on species (Patterson 1995), and a more rapid emergence of weed seedlings at an elevated CO2 has been documented under field conditions (Ziska and Bunce 1993). In common with pest management, the challenge for weed management research is to develop control strategies at a molecu- lar and crop management level that sustain and enhance farm profits while safeguarding the biological environ- ment and human health.
Climate change
Climate change is a contentious and complex issue; in rice science we would not wish to increase contribu- tions to potential climate change or avoid taking its possible consequences into account in our research. The two principal areas of rice research in rela- tion to climate change are: mitigation of greenhouse gas emissions from rice systems (Wassman et al. 2000) and discovering and adding adaptive fea- tures so that increases in production can continue if significant changes in climate occur (Sheehy et al. 2005a).
Rice fields are a source and a sink for environmentally significant gases (Khalil et al. 1990). Estimates of methane emissions from rice fields have improved as measurements across Asia have been made (Wassmann et al. 2000). Methane from rice accounts for about 10% of global methane emissions and in 2010 <http://www.globalmethane.org, 11th August 2011> was about 27.5 Mt CH4 yr-1 and emissions of N2O are probably less than 0.5% (by weight) of the value for methane.
Mid-season drainage in irrigated rice is one of the most effective tools for decreasing methane emissions and a single mid season aeration can reduce average seasonal emissions by 40% (Wassmann et al. 2010). However, N2O is released from drained fields and mid-season drainage must be managed so as not to induce yield depressions caused by water shortage.
Furthermore, the antagonism between CH4 and N2O emissions is a major impediment for devising effective mitigation strategies in the rice–wheat system: measures to reduce the emission of one GHG often intensify the emission of the other GHG (Wassmann et al. 2004).
In rainfed and deepwater rice, mitigation options are very limited in both num- ber and potential gains (Wassmann et al. 2000; Wassmann et al. 2009a; Wassmann et al. 2009b; Haefele et al. 2010). However, there is no reason to suspect that increasing grain yields will lead to an increase in CH4 emis- sions (Denier van der Gon et al. 2002).
Little thought has been given to the consequences of gaseous ammonia (NH3) emissions from rice. In many rice systems more than 50% of the nitrogen fertiliser applied can be lost through volatilisation (Vlek and Byrnes 1986; De Datta 1995). The total emis- sion of NH3 from rice can be estimat- ed theoretically from the current glob- al annual production of 690 million tonnes (593 million tonnes dry matter) containing 1.4% N plus 890 million tonnes of straw with 0.6% N.
Global rice biomass thus contains 13.6 million tonnes N, and an equal amount will have been lost by volatilisation from fertiliser, which is equivalent to 16.5 million tonnes NH3. The residence time of NH3 in the atmosphere is 10–30 d (Tsunogai and Ikeuchi 1968) and most of it is returned to the soil by precipitation and dry deposition within a few kilometres of its source. The effects of deposition are complex, but it can be damaging to natural veg- etation (Krupa 2003).
Over the past 25 years, minimum temperatures increased (0.04 °C yr-1) during both the dry and wet seasons at IRRI and maximum temperatures increased in the wet (0.02 °C yr-1), but not the dry season (Sheehy et al. 2005a). Over the 50 years of IRRI’s history atmospheric CO2 concentra- tions have risen from about 317 to 390 ppm (NOAA ESRL data). It has been shown that temperature increas- es in the range 22–32 °C reduced rice yields by 0.6 t ha-1 °C-1 (Baker and Allen 1993; Sheehy et al. 2006), whereas yield increases by about 0.5 t ha-1 for every 75 ppm increase in atmospheric CO2.
Overall, it is likely that over the next 50 years increases in daily average temperatures will have a greater negative effect on yield than the positive benefit of increasing CO2 (Sheehy et al. 2007a). However, the yield-depressing effects of high tem- peratures caused by weather extremes in the range 35–40 °C during flower- ing are a more serious threat to yield. Rice is self-pollinated and pollination normally occurs in late morning.
Permanent thermal damage to the reproductive mechanisms in that tem- perature range reduces grain yield by approximately 20% at 35 °C rising to 95% at 40 °C during flowering (Satake and Yoshida 1978; Horie et al. 1995; Prasad et al. 2006; Sheehy et al. 2005a, 2006). In theory, yield losses owing to infertility caused by high temperature during flowering can be minimised, but not completely elimi- nated, by changing the time of day at which the crop flowers to the cooler parts of the day (Sheehy et al. 2005a).
It is vital that clock genes be identified that allow rice to flower in the coolest part of the day or at night so as to avoid thermal infertility (Sheehy et al. 2007b). Other possible solutions are to discover mechanisms that confer toler- ance of high temperatures for vulnera- ble processes during the reproductive phase ( Prasad et al. 2006). Going from an El Niño year to a La Niña year in the Philippines decreases potential yield by 28% (Sheehy et al. 2005a); the damaging effects of weather extremes of all types are large (Table 1).
Developing rice resilient to climate change presents plant breeders with a substantial challenge; it should be storm-resistant, submergent-tolerant, flowering in early morning, salttoler- ant, fast-growing and high-yielding. The bioengineering of biological nitro- gen fixation into rice would reduce significantly the requirement for nitro- gen fertilisers (Fischer 2000) and sig- nificantly reduce ammonia volatilisation.
Managing rice to reduce gaseous emissions will remain important. Farmers must learn how to adapt to the consequences of climate change through choice of variety and appro- priate agronomy, which will be a chal- lenge and no easy matter.
Nutrients
Plant growth depends on the uptake of a range of mineral elements from the soil and increased yield depends on increased uptake. The large amounts of nutrients required in high yielding crops (Table 2) far exceeds the capacity of the soil to supply them without the application of fertilisers. The proportion of an applied fertiliser element that appears in the crop (fer- tiliser use efficiency for each mineral element) is highly variable depending on soil type and other environmental factors.
Thus, site specific nutrient management is recommended for increasing yield (Cassman et al. 1996; Olk et al. 1999; Dobermann et al. 2004). Of particular concern is the negative K balance in about 80% of the intensive rice fields in Asia. Dobermann et al. (1998) suggested that it was only a matter of time before the indigenous supply becomes a limiting factor on the most fertile complex and as new stress-tolerant cultivars are developed, novel nutrient management systems are required (Haefele and Hijmans, 2007; Haefele et al. 2009).
Greenwood et al. (1990) showed that C4 crops contained about 72% of the nitrogen of C3 crops at the same value of biomass. Mitchell and Sheehy (2006) estimated that the photosynthetic N- use efficiency of C4 rice would be about two and a half times greater than for C3 rice.
In rice, the rate at which the devel- oping panicle acquires N exceeds the rate at which the crop acquires it through its roots during grain filling (Sheehy et al. 1998). The requirement for a large N ‘reservoir’ in the leaf and other vegetative tissues was empha- sised by Sinclair and Sheehy (1999). Using the critical N model of Greenwood et al. (1990), crops can be managed to ensure that their N content is optimal for maximum yield.
However, yield potential is set by weather and the optimum N fertiliser required will be lower in a La Niña year than in an El Niño year. As an example, at IRRI, assuming 50% volatilisation, 415 kg N ha1 needs to be supplied to enable the critical N content in an El Niño year and 250 kg N ha1 in a La Niña year (Sheehy et al. 2005a,b).
How is a poor farmer in the developing world to access sea- sonal weather forecasts and predic- tions of optimum N rates? The quanti- ty of fertiliser N required to support maximum yields and the large losses into the environment lead to the con- clusion that replacing fertiliser N by biologically fixed N via a nodular sym-
biotic system should be a high priority for continued research (Sheehy et al. 2005b). However, the anaerobic envi- ronment of the roots of irrigated rice are not best suited to the oxygen requirements of root nodules and novel ways of water management in terms of raised beds may benefit attempts to nodulate rice roots.
Improving rice quality
The first semi-dwarf rice of the Green Revolution, IR8, did not have good grain quality but it demonstrated that high yields were possible. Apart from the importance to the consumer of desirable cooking and eating properties, it has been recognised that rice with concentrations of micronutrients higher than normal could make an effective contribution to reducing deficiencies in poor communities where rice forms a large part of the diet.
Biofortification has several compo- nents: breeding micronutrient-dense varieties of staple food crops; determining the availability to consumers of micronutrients in cooked foods and that they relieve deficiency; and ensur- ing general acceptability of new vari- eties to both farmers and consumers (Bouis et al. 2011).
The advantage of biofortification is that the benefits are enduring with no further inputs, whereas providing food supplements or fortification of food incurs continu- ing costs. Ultimately, economic devel- opment and diversification of diets will ensure adequate intake of micronutrients but until that occurs biofortification has a role to play.
Existing vari- eties, or landraces, with high concentrations of micronutrient must be sought or created by genetic engineer- ing, so that this attribute can be transferred to elite cultivars (adapted to local conditions, high-yielding, resistant to pests and diseases) using marker-assisted backcrossing.
Biofortification of rice is carried out at IRRI and in the HarvestPlus research programme of the CGIAR (www.har- vestplus.org) which has been operat- ing since 2004. Rice varieties with high concentrations of iron or zinc have been identified and used in breeding programmes. Rice high in zinc is expected to be released in India and Bangladesh in 2013.
Vitamin A is acquired direct from meat and dairy sources, but foods of plant origin provide ‚-carotene (pro- vitamin A) which is converted to vita- min A in the body. Rice plants pro- duce ‚-carotene (pro-vitamin A) in the green tissues but not in the edible part of the seed so natural variation is not available to be exploited in plant breeding.
Three genes for enzymes of the biochemical pathway for ‚- carotene were introduced to rice by genetic engineering (Ye et al. 2000). The ‚-carotene made in the grains gave a strong yellow colour prompting the name Golden Rice for this initia- tive. An improved version of Golden Rice with higher concentration of ‚- carotene was produced five years later (Paine et al. 2005).
Experiments have confirmed that the ‚-carotene in dietary rice is absorbed into the blood (Tang et al. 2009). The Golden Rice project at IRRI (www.irri.org/golden- rice) with partners in Bangladesh and the Philippines is using the transgenic rice in breeding programmes to pro- duce locally adapted varieties.
Data for safety and regulatory processes are being generated now and release to farmers should follow in several years. Rice contains little or no folate (B9). In recent years transgenic rice plants producing measurable amounts of folate have been produced, but this work is still at an experimental stage (Storozhenko et al. 2007).
C4 Rice
Carbon dioxide is first fixed into a compound in rice with three carbons (C3) by the photosynthetic enzyme ribulose bisphosphate carboxylase oxygenase (Rubisco) in mesophyll cells this is known as C3 photosynthesis (Fig. 3). Rubisco also reacts with oxygen (O2) which results in a cycle of reactions known as photorespiration (rather than photosynthesis) because there is uptake of O2 and output of CO2 as occurs in normal respiration.
At temperatures in excess of 20°C, O2 begins to out-compete CO2 and dramatic reductions in photosynthetic efficiency result. The C4 pathway has two spatially separated parts connected by a “CO2 pump”; the first part involves the initial fixation of CO2 into C4 acids using an enzyme that is insensitive to O2.
In the second part, CO2 is released from the C4 acid at a rate which creates a region of high CO2 concentration where it is fixed by Rubisco free of competition from O2. The build-up of CO2 by the “CO2 pump” requires extra energy from sunlight and therefore it is only in hot, sunny climates that the C4 pathway is beneficial.
Most C4 plants, and certainly all known C4 grasses, compartmentalise the two parts of the photosynthetic pathway between mesophyll (M) cells and bundle sheath (BS) cells which are morphologically distinct cell types that are arranged in concentric circles around veins (Nelson and Langdale 1992).
Enlarged BS cells surround the veins (V) and the BS cells are surrounded by M cells. Each pair of veins is thus separated by two bundle sheath and two mesophyll cells in a V- BS-M-M-BS-V pattern, as seen in transverse section of the leaf, referred to as Kranz anatomy. The twocell arrangement facilitates the development of a high concentration of CO2 in the BS cells which suppresses photorespiration.
The separation of metabolism is achieved by restricting the expression of a small number of genes to either the BS or M cells (Brown et al. 2005). Genes encoding all of these enzymes are present in C3 plants, but expressionis much lower than in C4 species. Despite the apparent biochemical and morphological complexity of the C4 mechanism, it is remarkably found independently in over 45 groups of unrelated species in 19 families of vascular plants including several different types of grass plant (Kellogg 1999).
This repeated presence of a complex trait indicates that it is relatively easy to generate. Indeed some plants are able to switch between C3 and C4 photosynthesis (Ueno 1998), indicating that the processes governing the function of these apparently complex systems must be flexible (Hibberd, et al. 2008).
The link between the efficiency of photosynthesis and yield was made by Monteith (1978) who compared the yields of a number of C3 and C4 crops growing over a range of crop dura- tions. He showed that C4 crops in sub- tropical and tropical climates could produce about 66% more biomass than C3 crops (Fig. 4).
The attraction of the full C4 system is not only its high yield, but also the doubled water- use efficiency and higher nitrogen-use efficiency that accompanies the trait. At the end of record breaking yield (~11 t ha1) experiments for IRRI in 1997 and 1998 with an elite indica and lines of a new plant type it was proposed that further yield increases were probably beyond the capacity of rice with its C3 photosynthetic system (Sheehy et al. 2000).
Mitchell and Sheehy (1998) investigated radiation use efficiency (defined by Monteith, 1977) and showed that it was strongly related to photosynthesis. The hypothesis that an increase in radia- tion use efficiency (RUE) would result in an increase in yield was tested experimentally.
Rice and maize were grown simultaneously at IRRI in 2006 without limitations of water and nutri- ents and the yields were 8.3 tha1 and 13.9 t ha1; the RUE values were 2.9 g DW MJ1 and 4.4 g DW MJ1, respectively (Sheehy et al. 2007a). It was recognised that to increase maximum yields by 50%, the RUE of rice would have to rise to that of maize and to make that possible the C3 photosyn- thetic system of rice would have to be converted to a C4 system (Mitchell and Sheehy 2006; Sheehy et al. 2008).
Although controversial to some peo- ple, genetic engineering has proved indispensable for transferring genes between sexually incompatible species to produce transgenic plants for agriculture (Mitchell and Sheehy 2000). Furthermore, attempts have been made to engineer novel multigene pathways to increase photosynthesis in leaves (Suzuki et al. 2006) and to recapture CO2 from photorespiration (Kebish et al. 2007).
Arabidopsis is often used as a tool for understanding the molecular biology of the plant partly because it has a small and well documented genome. Brown et al. (2005) suggested using Cleome gynandra, the C4 plant most closely related to Arabidopsis, to accelerate an understanding of the anatomical aspects of the C4 syndrome.
However, when considering such a radical change to the photosynthetic system the possibility of confounding factors such as resource rejection by plants is often overlooked (Thomas and Sadras 2001). Also not all plants are able to up-regulate their photosynthetic activity when grown at high concentrations of CO2 (Allen 1994) indicating there may be sink limitations which feed back to prevent increases in photosynthesis.
Ingram et al. (1997) and Baker and Allen (1993) concluded that rice photosynthesis and yield did not increase at CO2 concentrations above 500 ppm. It is of vital importance to consider the rela- tive importance of source strength and sink capacity when programmes aimed at increasing yields are planned. Harvest index (HI) relates grain yield and biomass, with grain yield being expressed as a proportion of the total above-ground dry matter.
Sheehy et al. (1998) showed that the HI of well managed rice crops was nearly con- stant at 0.5 and so improvements in yield via improvements in HI were most unlikely. A C4 rice crop must have a larger vegetative biomass to deliver a larger grain yield.
How could C4 rice be constructed? Let us consider what type of rice might have the sink capacity to use a supercharged photosynthetic apparatus in order to increase substantially both photosynthesis and growth rate. Rice is a weak perennial with two strong phases of logistic growth: vege- tative growth followed by reproduc- tive growth (Sheehy et al. 2004).
First let us deal with the vegetative phase of growth and imagine that it is possi- ble to increase the maximum grain yields of irrigated rice growing over 100 days in the tropics by 50% e.g. to about 15 t ha-1. Given a HI of 0.5, the total vegetative biomass would have to increase by 50%, therefore the masses of leaves and stems would have to increase by the same amount.
The HI concept provides no indication of the individual units of production, the tillers, but stem mass and stem strength (to prevent lodging) would have to increase. However, it is clear that the Green Revolution varieties of dwarf indica rice do not possess the stems that characterize maize, sorghum or many of the wild relatives of rice. Consequently, it is unlikely that dwarf indicas could produce the required increase in stem biomass and therefore the 50% increase in vegeta-tive biomass needed for C4 rice.
Furthermore, we speculate that the suppression of the stem sink for assimilation is what ultimately limits the up- regulation of photosynthesis in response to increasing CO2 concentra- tions in dwarf indicas. Indeed, we sug- gest that the wild relatives of rice and landraces should be screened for the ability to up-regulate leaf photosyn- thesis at high concentrations of CO2.
In contrast, in the reproductive phase, rice has unused sink capacity for spikelet development and the evidence (Sheehy et al. 2001b) indicates that less than half the available spikelets are converted into grain. This is why in experiments with elevated CO2 concentrations increases in grain yield were observed (Baker and Allen 1993; Ziska et al. 1997; Kobayashi et al. 2005). Sink strength has two components and it is the vegetative, rather than the reproductive one that requires additional attention.
Let us now turn to the issues sur- rounding source strength. The rapid development of molecular tools and technologies encouraged IRRI to invest in a ‘high-risk high-return’ C4 rice project. The first priority was to build a team of multidisciplinary partners with complementary skills from advanced institutions across the world and so IRRI formed a Global Consortium for C4 rice.
Once formed, the Consortium agreed a strategy, which was to install a maize-like pho- tosynthesis mechanism in rice. The tactics and operational approaches would centre on discovering the cas- sette of genes necessary to make this a reality. Given the complexity of the required genetic transformation, it was thought unwise at the early stage to prioritise a single research route to developing C4 rice and the consortium decided to pursue several paths in parallel.
The objectives can be divided into two groups as shown in Fig. 5: those involving gene discovery (direct) and those concerning technical devel- opments underpinning the direct approaches (support).
It was obvious from the outset that there was a lack of understanding of the genetic control of leaf develop- ment, particularly the key feature of close vein spacing—the starting point for Kranz anatomy. To fill this gap a number of approaches are being actively pursued.
- The development of screening tools for changes in leaf anatomy and CO2 compensation point.
- Mutagenising sorghum, a C4 plant, and screening for C3-like reversions.
- Using molecular tools to investigate cell-specific aspects of maize husk leaves which have leaf.anatomy and carbon fixation more like rice and
- that differ from foliar leaves which have Kranz anatomy and C4 function .
- Establishing transcriptome atlases of maize, sorghum and rice leaf meso- phyll and bundle sheath cells and using it as the foundation for a sys- tems biology approach to the under- standing of photosynthetic develop- ment and the discovery of C4 genes (Pinghua et al. 2010).
- Screening available rice activation tagging lines for C4-like traits.
- Screening mutants of the rice culti- var IR64 for any tendency towards C4 characteristics.
- A molecular tool box is being built that allows the expression of genes specifically in mesophyll cells (M) or bundle sheath cells (BS) of rice.
- Molecular approaches used to gen- erate a comprehensive understanding of which maize proteins are needed for the C4 pathway to be active in M or BS cells of rice.
- To make lines of rice containing substantial parts of the C4 pathway. This will determine whether compart- mentation of biochemistry required for C4 photosynthesis into M and BS cells leads to alterations in leaf anatomy associated with the pathway.
- To develop a set of promoters of varying strength and specific for M or BS cells of rice.
- Apply bioinformatics methodology in support of gene discovery.
- To search for wild rice types con- taining any tendency towards C4 char- acteristics.
- To use wild types to uncover genes crucial in the formation of a suitable platform for the expression of a C4 rice cassette of genes.
The timeline of the C4 initiative can be conveniently divided into three phases that are estimated at current rates of funding to demand 15 years of coordinated research carried out at IRRI and in the laboratories of the C4 Rice Consortium. Phase I is largely about proving the concept and assem- bling the components required to construct C4 rice. Phase II is about constructing prototypes of C4 rice using the genes, tools and knowledge generated in Phase I. Phase III will be concerned with optimising C4 rice in locally adapted cultivars and delivering its benefits to consumers.
Funding
The success of the first Green Revolu- tion over the past three decades diverted the world’s attention from agriculture. This resulted in lower support for agricultural research so that for some centres, such as the International Rice Research Institute (IRRI), the downward trend in funding continued until 2006 with the budget
in real dollars falling to the 1978 fig- ure. Furthermore, developed nations reduced their investments in agricultural research and turned their remain- ing research investments away from productivity gains (Pardey et al. 2006). The slowing in the rate of increase in rice production has been accompanied by a doubling of rice prices during the past five years.
Sheehy (2003) calculated the benefit accruing from a C4 rice replacing C3 rice could be as high as 43.7 billion US dollars a year; that figure would now be closer to 100 billion US dollars. Furthermore, Sheehy et al. (2007c) suggested that the money spent annually on research aimed at preventing malaria or HIV/AIDS far exceeds that spent each year on cut- ting edge research such as C4 rice despite 25,000 people dying each day from causes related to hunger. Signifi- cant progress in yield enhancement research will require the integration of efforts from those engaged in funda-
mental and applied research. All forms of research require a funding flow and it is only in the more affluent societies that curiosity-driven research can pros- per. Curiosity and demand for products are the engines of research and one form of research can stimulate the other.
However, funding mechanisms to integrate the research required for C4 rice, across national and disciplinary boundaries, are almost non-existent with the exception of that of the Bill & Melinda Gates Foundation. Since 2000, the funding situation has improved for the CGIAR Centres, but almost all the increase comes from grants earmarked for specific research projects. This trend is to be regretted as it seems it will not be possible for the Centres to embark on the innova- tive, risky but visionary research which, if successful, is most likely to transform agricultural productivity in the face of climate change.
Conclusions
The breeding of semi-dwarf cultivars in the 1960s was an example of transformative research: a change of plant height, unimportant in itself, led to substantially higher yields because the plants could take up more nitrogen and carry heavier panicles without lodging.
This made investments in irrigation and crop protection worthwhile. The cultivars of the Green Revolution more than doubled the food supply in Asia in 25 years, with an increase of only 4% in net cropped area (Lipton 2007; Rosegrant and Hazell 2000). However, population growth is generating a demand for increased grain production that is beyond the delivery capacity of the current rice genome.
The tools of molecular biology pro- vide us with opportunities unavail- able to plant breeders of the past: they enable us to identify the genes responsible for desirable traits (such as SUB1) and then to transfer them efficiently into new cultivars. The tools can also be used to transfer genes between sexually incompatible species such as maize and rice.
A maize-like C4 photosynthetic path- way in rice would have the potential to deliver a very large improvement in yield; it would double water-use efficiency and increase nitrogen-use efficiency (Sheehy and Mitchell 2006). The combination of advan- tages peculiar to C4 rice means the syndrome could be used in all of the
rice ecosystems to improve yields. It is easy to suggest that the construc- tion of C4 rice will be especially diffi- cult given current knowledge, or that the cost might be unusually high for agricultural research. But the need is correspondingly high: global popula- tion continues to increase, climate change will alter cropping patterns and probably reduce yields, and water available for agriculture will become scarce or more expensive.
Constructing C4 rice is a high- reward, high-risk venture, and with current funding is likely to take about 15 years to complete. It will require the ingenuity and skills of researchers from institutions in many countries, hence the formation of a Consortium for C4 Rice.
Future climates are expected to be more variable with frequent episodes of high temperatures during the growing season (Prasad et al. 2006). High temperature during flowering decreases spikelet fertility and yield. However, there is poten- tial for genetic improvement of heat tolerance and for changing the time of flowering to the cooler parts of the day. Breeding rice crops that are heat tolerant must continue to be a priority.
Protecting yields from losses caused by pests, diseases and weeds means integrated pest man- agement and weed research need to benefit from continuing research investment. The genetic diversity of rice is conserved in the IRRI Gene Bank and it provides researchers with germplasm for screening. It is a highly valued asset without which progress in many research projects could not be made.
Farmers have to deliver the benefits of new science, yet in the developing world crops are often grown on land areas of a few hectares, or less, using the simplest of tools and traditional knowledge. High performance technologies, such as the C4 syndrome or submergence toler- ance, delivered in seeds would give
poor farmers an opportunity to improve their livelihoods without re- education or the need for other equipment. The new management systems that could help mitigate the emission of greenhouse gases require education and access to information produced by weather forecasts and predictive modeling tools. To take advantage fully of such opportunities there is a need to develop and deliver decision support systems for crop management for which the growing and wide- spread use SMS text on mobile phones and specialized ‘Apps’ offer great potential.
In our opinion at least half of the budget of the CGIAR should be directed to transformative research aimed at producing a large increase in the yields of all its agricultural systems in an environmentally accept- able manner. Biofortification is also another area which can be system- wide and has the potential to make a permanent and widespread change in rice quality. The newly formed Global Rice Science Partnership (GRiSP) has 900 research partners worldwide and represents, for the first time, a single strategic plan for global rice research. It remains to be seen if this
and similar initiatives will generate even more acronyms and largely fund low-risk research or stimulate the investment needed for the high- risk, innovative and transformative science needed to tackle the mas- sive problems discussed in this paper.
The economic benefits that would flow from rice, wheat and legumes using supercharged photosynthesis and biological nitrogen fixation would be many billions of dollars annually (Sheehy 2003). The cost of discovering the cassettes of genes responsible for both of those syn- dromes would probably be of the order of a several hundred million dollars; the cost-benefit ratio is enor- mous. It must be remembered that expensive is different to impossible.
Acknowledgements
We are grateful to Anaida Ferrer at IRRI for preparing figures for publi- cation and to Gene Hettel for allow- ing us access to the photographic resources at IRRI. Use of facilities at the Department of Animal and Plant Sciences and support from Professor F.I. Woodward are gratefully acknowledged by P.L.M.
References
- Allen, L.H. Jr. (1994) Carbon Dioxide Increase: Direct impacts of crops and indirect effects medi- ated through anticipated climate changes. In Physiology and Determination of crop Yield. Eds. I K.J. Boote, J.M. Bennett, T.R. Sinclair and G.M. Paulsen. ASA-CSSA-SSA: Maidison, Wisconsin. pp. 425-459.
- Allen Jr., L.H., Vu, J.C.V. and Sheehy, J. (2003) Carbon dioxide, plants and transpiration. In Encyclopedia of Water Science. Eds. S.W. Trimble, I B.A. Stewart and T.A.Howell. Taylor and Francis. pp. 57-61.
- Aimrun, W., Amin, M.S.M. and Gholizadeh, A. (2010) Spatial Variability of Irrigation Water Percolation Rates and its Relation to Rice Productivity. American Journal of Applied Sciences, 7 (1), 51-55.
- Bailey-Serres, J., Voesenek, LA.C. (2008) Flooding stress: acclimations and genetic diversity. Ann Rev Plant Biol. 59, 313–39.
- Baker, J.T. and Allen, Jr., L.H. (1993) Effects of CO2 and temperature on rice: a summary of five growing seasons. J. Agric. Meteorol. 48, 575-582. I Bouis, H.E., Hotz, C., McClafferty, B., Meenakshi, J.V. and Pfeiffer, W.H. (2011) Biofortification: A new tool to reduce micronutri- ent malnutrition. Food and Nutrition Bulletin 32: Supplement 1, 31S-40S.
- Bouman, B.A.M. and Tuong, T.P., (2001) Field water management to save water and increase its productivity in irrigated lowland rice. Agric. Water Management. 49, 11–30.
- Bouman, B., Barker, R., Humphreys, E. and Tuong, T.P. 2007. Rice: feeding the billions. In Water for Food, Water for Life. Ed. D. Molden. London (Earthscan). pp. 515–549.
- Brown, N., Parsley, K. and Hibberd, J.M. (2005) The future of C4 research— maize, flaveria or cleome? Trends Plant Sci. 10, 215-221.
- Cassman, K.G., Dobermann, A., Sta Cruz, P.C., Gines, G.C., Samson, M.I., Descalsota, J.P., Alcantara, J.M., Dizon, M.A. and Olk, D.C. (1996) Soil organic matter and the indigenous nitrogen supply of intensive irrigated rice systems in the tropics. Plant and Soil 182, 267-278.
- Catling ,D. (1992) Rice in deep water. London: MacMillan Press Ltd.
- Chapagain, A.K. and Hoekstra, A.Y. (2011) The blue, green and grey water footprint of rice from production and consumption perspectives, Ecological Economics, 70 (4): 749-758.
- Connor, D.J., Jones, T.J. and Palta, J.A. (1985) Response of sunflower to strategies of irrigation. I. Growth, yield and the efficiency of water use. Field Crops Research, 10, 15-26.
- Dawe, D. (2000) The contribution of rice research to poverty alleviation. In Redesigning rice photosynthesis to increase yield. Eds. J.E. Sheehy, P.L. Mitchell and Hardy B. (2000) Proceedings of the Workshop on The Quest to Reduce Hunger: Redesigning Rice Photosynthesis, 30 Nov.-3 Dec. 1999, Los Baños, Philippines. Makati City (Philippines): International Rice Research Institute and Amsterdam (The Netherlands): Elsevier Science B.V. pp. 293.
- De Datta, S.K., (1981) Principles and Practices of Rice Production. IRRI, Los Baños, Philippines. pp. 618.
- De Datta, S.K. (1995) Nitrogen transformations in wetland rice ecosystems. Fert. Res. 42, 193- 203.
- Denier van der Gon, H.A.C., Kropff, M.J., van Breemen, N., Wassmann, R., Lantin, R.S., Aduna,E.A., Metra-Corton, T., and van Laar, H.H. (2002) Optimising grain yields reduces CH4 emis- sions from rice paddy fields. Proceedings of the National Academy of Sciences USA 99, 12021- 12024. de Wit, C.T. (1958) Transpiration and crop yields. Versl. Landbouwk. Onderz. No. 64.6. 88 p. I Dobermann, A., Cassman, K.G., Mamaril, C.P. and Sheehy, J.E. (1998) Management of phospho- rus, potassium, and sulfur in intensive, irrigated lowland rice. Field Crops Research 56, 113-138. I Dobermann, A., Abdulrachman, S., Gines, H.C., Nagarajan, R., Satawathananont, S., Son, T.T., Tan, P.S., Wang, G.H., Simbahan, G., Adviento, M.A. and Witt, C. (2004) Agronomic performance of site-specific nutrient management in intensive rice cropping systems in Asia. In Increasing the productivity of intensive rice systems through site- specific nutrient management. Eds. A. IDobermann, C. Witt and D. Dawe. Enfield, N.H. (USA) and Los Baños (Philippines): Science Publishers, Inc., and International Rice Research Institute (IRRI). pp. 307-336.
- FAOSTAT. (2011) Website for data on world crop production (updated 17th May 2011), accessed on 30th June 2011, at http://faostat.fao.org/site/567/default.aspx.
- Fischer, K.S. (2000) Frontier project on nitrogen fixation in rice: looking ahead. In The quest for nitrogen fixation in rice. Eds. J.K. Ladha and P.M. Reddy. Proceedings of the Third Working Group meeting on assessing opportunities for nitrogen fixation in rice, 9-12 Aug. 1999. International Rice Research Institute, Laguna, Philippines, pp. 25-31. I Foresight (2011) The Future of Food and Farming: Challenges and Choices for Global Sustainability. London (Government Office for Science).
- Fukao, T. and Bailey-Serres, J. (2008a) Ethylene-a key regulator of submergence respons- es in rice. Plant Sci. 175, 43-51.
- Godfray, H.C.J., Beddington, J.R., Crute, I.R., Haddad, L., Lawrence, D., Muir, J.F., Pretty, J., Robinson, S., Thomas, S.M. & Toulmin, C.
- (2010) Food security: the challenge of feeding 9 billion people. Science 327: 812–818.
- Greenwood, D.J., Lemaire, G., Gosse, G., Cruz, P., Draycott, A., Neeteson, J.J. (1990) Decline in percentage N of C3 and C4 crops with increasing plant mass. Annals of Botany 66, 425-436.
- GRiSP (2010) Global Rice Science Partnership. Available at http://irri.org/our-science/global-rice- science-partnership-grisp (accessed on 8th July 2011).
- Guerra, L.C, Bhuiyan, S.I., Tuong, T.P. & Barker, R. (1998) Producing more rice with less water from irrigated systems. SWIM Paper No. 5, Colombo, International Water Management Institute.
- Haefele, S.M. and Hijmans, R.J. (2007) Soil quality in rice-based rainfed lowlands of Asia: characterization and distribution. In Science, tech- nology, and trade for peace and prosperity. Eds. P.K. Aggarwal, J.K. Ladha, R.K. Singh, C. Devakumar and B. Hardy. Proceedings of the 26th International Rice Research Conference, 9-12 Oct 2006, New Delhi, India. Los Baños (Philippines): International Rice Research Institute, Indian Council of Agricultural Research, and National Academy of Agricultural Sciences. pp. 297-308.
- Haefele SM, Knoblauch C, Gummert M, Konboon Y, Koyama S. (2009) Black carbon (biochar) in rice-based systems: characteristics and opportunities. In Amazonian dark earths: Wim Sombroek’s vision. Eds. W.I. Woods, W.G. Teixeira, J. Lehmann, C. Steiner, A. WinklerPrins, L. Rebellato, Springer Science + Business Media B.V. pp. 445-463.
- Haefele, S.M., Ismail, A.M., Johnson, D.E. , Vera Cruz, C. and Samson, B. (2010) Crop and natural resource management for climate-ready rice in unfavorable environments: coping with adverse conditions and creating opportunities. http://irri.org/climatedocs/presentation_Lists/Docs /2_Haefele.pdf
- Hattori, Y. Nagai, K., Furukawa, S., Song, X.J., Kawano, R., Sakakibara, H., Wu, J.Z., Matsumoto, T., Yoshimura, A., Kitano, H., Matsuoka, M.,
- Mori, H. and Ashikari, M. (2009) The ethylene response factors SNORKEL1 and SNORKEL2 allow rice to adapt to deep water. Nature 460, 1026- 1031.
- Heong, K.L. and Hardy, B. (2008) Planthoppers – New threats to the sustainability of intensive rice production systems in Asia. International Rice Research Institute, Los Banos, Philippines. pp. 460.
- Heong, K.L., Escalada, M.M., Huan, N.H. and Mai, V. 1998. Use of communication media in changing rice farmers' pest management in South Vietnam. Crop Protection, 17 (5), 413 – 425.
- Hibberd, J.M., Langdale, J.A. and Sheehy, J. (2008) Using C4 photosynthesis to increase the yield of rice-rationale and feasibility. Current Opinion in Plant Biology 228-231.
- Horie, T., Nakagawa, H., Centeno, H.G.S.and Kropff, M.J. (1995) The rice crop simulation model SIMRIW and its testing. In Modeling the impact of climate change on rice production in Asia. Eds. Matthews R.B., Kropff, M.J., Bachelet, D. and van Laar, H.H. Wallingford (UK): CAB International, and Manila (Philippines): International Rice Research Institute. pp. 51-66. Ingram, K.T., Manalo, P.A. and Pamplona, R.R. (1997) Carbon dioxide and temperature: effects on Growth and development of rice cultivars. In Advances in carbon dioxide effects research ASA Special Publication no. 61.
- Jimenez, R.R., and Ladha, J.K. (1993) Automated elemental analysis: a rapid and reli- able but expensive measurement of total carbon and nitrogen in plant and soil samples. Commun. Soil Sci. Plant Anal. 24 (15&16):1897-1924.
- Kebeish R, Niessen M, Thiruveedhi K, Bari R, Hirsch H-J, Rosenkranz R, Stäbler N, Schönfeld B, Kreuzaler F, Peterhänsel C. (2007). Chloroplastic photorespiratory bypass increases photosynthesis and biomass production in Arabidopsis thaliana. Nature Biotechnology 25, 593-599.
- Kellogg, E.A. (1999) Phylogenetic aspects of the evolution of C4 photosynthesis. In C4 Plant Biology. Eds. Sage R.F. and Monson R.K. Academic Press; pp. 411-444.
- Khalil, M.A.K., Rasmussen, R.A., and Wang M.X. (1990) Emissions of Trace Gases from Chinese Rice Fields and Biogas Generators: CH4, N2O, CO, CO2, Chlorocarbons and Hydrocarbons. Chemosphere 20, 207-226.
- Kobayashi, K., Kim, H., Lieffering, M., Miura, S. and Okada, M. (2005) Paddy rice response to free-air CO2 enrichment. Journal of Agricultural Meteorology 60, 475–479.
- Kropff, M.J., Cassman, K.G., Peng, S., Matthews, R.B., and Setter, T.L. (1994) Quantitative understanding of yield potential. In Breaking the Yield Barrier. Ed. Cassman, K. G. Proceedings of a Workshop on Rice Yield Potential in Favorable Environments, International Rice Research Institute, Philippines, pp. 21-38.
- Krupa, S. V. (2003). Effects of atmospheric ammo- nia (NH3) on terrestrial vegetation: A review. Environmental Pollution, 124, 179–221.
- Latshaw, W.L. and Miller, E.C. (1924) Elemental Composition of the Corn Plant. J. Agric. Res. 27 (11),845-861.
- Lipton, M. (2007) Plant breeding and poverty: Can transgenic seeds replicate the 'green revolu- tion' as a source of gains for the poor? Journal of Development Studies 43, 31-62.
- Mackill, D.J., Amante, M.M., Vergara. B.S. and Sarkarung. S. (1993) Improved semidwarf rice lines with tolerance to submergence of seedlings. Crop Sci. 33, 749–75.
- Mackill , D.J., Coffman, W.R. and Garrity, D.P. (1966) Rainfed lowland rice improvement. Los Banos, The Philippines: International Rice Research Institute.
- Mackill, D.J. (2006) Breeding for resistance to abiotic stresses in rice: the value of quantitative trait loci. In Plant breeding: the Arnel R Hallauer International Symposium Ames. Eds. Lamkey K.R. and Lee M. Blackwell Publishing. pp. 201–12.
- Mishra, S.B., Senadhira, D. And Manigbas, N.L. (1996) Genetics of submergence tolerance in rice (Oryza sativa L.). Field Crops Res. 46,
- 177–81.
- Mitchell, P.L., Sheehy, J.E. and Woodward, F.I. (1998) Potential yields and the efficiency of radi- ation use in rice. IRRI Discussion Paper Series No. 32. Manila (Philippines). pp. 62.
- Mitchell, P.L. and Sheehy, J.E. (2000) Genetic modification and agriculture. In Redesigning rice photosynthesis to increase yield. Eds. J.E. Sheehy, P.L. Mitchell and B. Hardy. (2000) Proceedings of the Workshop on The Quest to Reduce Hunger: Redesigning Rice Photosynthesis, 30 Nov.-3 Dec. 1999, Los Baños, Philippines. Makati City (Philippines): International Rice Research Institute and Amsterdam (The Netherlands): Elsevier Science B.V. pp. 293.
- Mitchell, P.L. and Sheehy, J.E. (2006) Supercharging rice photosynthesis to increase yield. New Phytologist 171, 688–693.
- Monteith, J.L. (1977) Climate and the efficiency of crop production in Britain. Philos. Trans. Royal Soc. London B, 277-294.
- Monteith, J.L. (1978) Reassessment of maxi- mum growth rates for C3 and C4 crops. Experimental Agriculture 14, 1-5.
- Nelson, T., Langdale, J.A. (1992) Developmental genetics of C4 photosynthesis. Ann. Rev. Plant Physiol. Plant Mol. Biol. 43, 25-47. Olk, D.C., Cassman, K.G., Simbahan, G., Sta. Cruz, P.C., Abdulrachman, S., Nagarajan, R., Pham Sy, T. and Satawathananont, S. (1999) Interpreting fertilizer-use efficiency in relation to soil nutrient-supplying capacity, factor productivi- ty, and agronomic efficiency. Nutrient Cycling in Agroecosystems 53, 35-41.
- Paine, J.A., Shipton, C.A., Chaggar, S., Howells, R.M., Kennedy, M.J., Vernon, G., Wright, S.Y., Hinchliffe, E., Adams, J.L., Silverstone, A.L. and Drake, R. (2005) A new version of Golden Rice with increased pro-vitamin A content. Nature Biotechnology 23, 482-487.
- Pardey, P.G., Alston, J.M. and Piggott, R.R. (eds) (2006) Agricultural R&D in the developing world. International Food Policy Research Institute, Washington, D.C. pp. 372
- Patterson, D. T. (1995). Weeds in a changing climate. Weed Sci. 43, 685–701.
- Penman, H.L. (1948) Natural evaporation from open water, bare soil, and grass. Proc. R. Soc. Lond. A193, 120-45.
- Pinghua , P., Ponnala, L., Gandotra, N., Wang, L., Si, Y., Tausta, S.L., Tesfamichael, H.K., Provart, N., Patel, R., Myers, C.R., Reidel, E.J., Turgeon, R., Liu, P., Sun, Q., Nelson,T. and Brutnell, T.P. (2010) The developmental dynamics of the maize leaf transcriptome. Nature Genetics, 42, 1060- 1067.
- Prasad, P.V.V., Boote, K.J., Allen, L.H., Jr., Sheehy, J.E. and Thomas, J.M.G. (2006) Species, ecotype and cultivar differences in spikelet fertility and harvest index of rice in response to high tem- perature stress. Field Crops Research 95, 398-411. I Rice Almanac. (2002) Rice Almanac (third edi- tion). Manila, Philippines (International Rice Research Institute; edited by J.L. Maclean, D.C. Dawe, B. Hardy and G.P. Hettel. pp. 253.
- Rodenburg, J., Meinke, H., Johnson, D. (2011) Challenges for weed management in African rice systems in a changing climate. J. Agric. Science (Cambridge), 149, 427-435.
- Rosegrant, M. and Hazell, P. (2000) Transforming the rural Asian economy: The unfin- ished revolution. Oxford University Press, Hong Kong, for the Asian Development Bank. pp. 512. I Royal Society. 2009. Reaping the Benefits: Science and the Sustainable Intensification of Global Agriculture. London (The Royal Society; Policy Document 11/09).
- Rubia, F.G. and Penning de Vries, F.W.T. (1990) Simulation of rice yield reductions caused by stem borer (SB). Int. Rice Res. Newsl. 15 (1), 34-35.
- Satake, T. and Yoshida, S. (1978) High temper- ature-induced sterility in indica rices at flowering. Jpn. J. Crop Sci. 47 (1), 6-17.
- Shashidhar, H.E. (2007) Aerobic rice –An effi- cient water management strategy for rice produc- tion. In Food and Water Security Eds. U. Aswathanarayana, Taylor & Francis. pp. 360.
- Sheehy, J.E., Dionora, M.J.A., Mitchell, P.L., Peng, S., Cassman, K.G., Lemaire, G. and Williams, R.L. (1998) Critical nitrogen concentra- tions: implications for high yielding rice (Oryza sativa L.) cultivars in the tropics. Field Crops Res. 59, 31-41.
- Sheehy, J.E., Mitchell, P.L,. Dionora, M.J.A., Tsukaguchi, T., Peng, S. and Khush, G.S. (2000) Unlocking the yield barrier in rice through a nitro- gen-led improvement in the radiation conversion factor. Plant Prod. Sci. 3, 372-374.
- Sheehy, J.E. (2001a). Will yield barriers limit future rice production? In Crop science: progress and prospects. Eds. J. Nösberger, H.H. Geiger and P.C. Struik. Hamburg (Germany): CAB International. pp. 281-305.
- Sheehy, J.E., Dionora, M.J.A. and Mitchell, P.L. (2001b) Spikelet numbers, sink size and potential yield in rice. Field Crops Res. 71, 77-85.
- Sheehy, J.E. (2003) Plant design: concepts and constraints. Indian J. Plant Physiol. Special Issue. pp. 36-48.
- Sheehy, J.E., Mitchell, P.L. and Ferrer, A.B. (2004) Bi-phasic growth patterns in rice. Ann. Bot. 94, 811-817.
- Sheehy, J., Elmido, A.E., Centeno, G. And Pablico, P. (2005a) Searching for new plants for climate change. J..Agric. Meteorol. 60 (5), 463- 468.
- Sheehy, J.E., Mitchell, P.L., Kirk, G.J.D. and Ferrer, A.B. (2005b) Can smarter nitrogen fertiliz- ers be designed? Matching nitrogen supply to crop requirements at high yields using a simple model. Field Crops Res. 94, 54-66.
- Sheehy, J.E., Mitchell, P.L., Allen, L.H. and Ferrer, A.B. (2006) Mathematical consequences of using various empirical expressions of crop yield as a function of temperature. Field Crops Res. 98, 216-221.
- Sheehy, J.E., Ferrer, A.B., Mitchell, P.L., Elmido- Mabilangan, A., Pablico, P. and Dionora, M.J.A . (2007a) How the rice crop works and why it needs a new engine. In Charting new pathways to C4 rice. Eds. J.E. Sheehy, P.L. Mitchell and B. Hardy. International Rice Research Institute, Los Baños, Philippines. pp. 3-26.
- Sheehy, J.E., Mabilangan, A.E., Dionora, M.J.A . and Pablico, P. (2007b) Time of day of flowering in wild species of the genus Oryza. International Rice Research Notes, 32 (1), 12-13.
- Sheehy, J.E., Ferrer, A.B, and Mitchell, P.L . (2007c): Harnessing photosynthesis in tomorrow’s world: Humans, crop production and poverty alle- viation. In Energy from the sun. Proceedings of the 14th International Congress of Photosynthesis Glasgow. Eds. J.F. Allen, E. Gantt, J.H. Golbeck
- and B. Osmond. Heidelberg: Springer. pp. 1237- 1242.
- Sheehy, J.E., Gunawardana, D., Ferrer, A.B., Danila, F., Tan, K.G. and Mitchell, P.L. (2008) Systems biology or the the biology of systems: routes to reducing hunger. New Phytologist 179, 579–582.
- Sheehy, J.E. and Mitchell, P.L. (2011). Rice and global food security: the race between scientific discovery and catastrophe. In Access not Excess,
- Eds. C. Pasternak. Smith-Gordon & Co. ISBN 9- 781-85463-245-6. pp. 81-90.
- Sinclair, T.R. and Sheehy, J.E. (1999) Erect leaves and photosynthesis in rice. Science 283, 1456-1457.
- Stanners, D. and Bourdeau, P. (1995) Europe's environment. Statistical compendium for the Dobris assessment. ECSC-EC-EAEC, Brussels and Luxembourg.
- Storozhenko, S., De Brouwer, V., Volckaert, M., Navarrete, O., Blancquaert, D., Zhang, G.- F.,Lambert, W., Van Der Straeten, D. (2007) Folate fortification of rice by metabolic engineering. Nat. Biotechnol. 25, 1277-1279.
- Suzuki, S., Murai, N., Kasaoka, K., Hiyoshi, T., Imaseki, H., Burnell, J.N. and Arai, M. (2006) Carbon metabolism in transgenic rice plants that express phosphoenolpyruvate carboxylase and/or phosphoenolpyruvate carboxykinase. Plant Science 170, 1010-1019.
- Tabbal, D. F., Bouman, B. A. M., Bhuiyan, S. I., Sibayan E. B. and Sattar, M. A. (2002) On farm strategies for reducing water input in irrigated rice; case studies in the Philippines. Agricultural Water Management. 56 (2), 93-112.
- Tang, G., Qin, J., Dolnikowski, G.D., Russell, R.M. & Grusak, M.A. (2009) Golden Rice is an effective source of vitamin A. American Journal of Clinical Nutrition 89, 1776–1783.
- Tanner, C.B. and Sinclair, T.R. (1983) Efficient water use in crop production: research or re- search?. In Limitations to efficient water use in crop production. Eds. H.M. Taylor, W.R. Jordan and T.R. Sinclair. Am. Soc. Agron., Madison, WI. pp. 128.
- Thiyagarajan, T.M. (2001) Water-saving rice cultivation in India. In Water-saving rice produc- tion systems. Eds. H. Hengsdijk and P.S. Bindraban. Proceedings of an international sym- posium on water-saving rice production systems at Nanjing University, China, April 2-4, 2001. pp. 15-45.
- Thomas, H. and Sadras, V.O. (2001) The cap- ture and gratuitous disposal of resources by plants. Functional Ecology 15, 3–12.
- Tsunogai, S. and Ikeuchi, K. (1968) Ammonia in the atmosphere. The Geochemical Society of Japan, Geochemical Journal 2, 157-166.
- Ueno, O. (1988) Induction of Kranz anatomy and C4-like biochemical characteristics in a sub- merged amphibious plant by abscisic acid. Plant Cell 10, 571-583.
- Vergara, B.S., and Mazaredo, A. (1975) Screening for resistance to submergence under greenhouse conditions. In Proceedings of the International Seminar on Deepwater Rice August 1974. Bangladesh Rice Res. Inst., Dhaka,
- Bangladesh. pp.67-70.
- Vlek, P.L.G. and Byrnes, B.H. (1986) The effica- cy and loss of fertilizer N in lowland rice. Fert. Res. 9, 131-147.
- Wassmann, R., Lantin, R.S., Neue, H.U., Buendia, L.V., Corton, T.M. and Lu, Y. (2000) Characterization of methane emissions from rice fields in Asia. III. Mitigation options and future research needs. Nutr. Cycl. Agroecosyst. 58, 23- 36.
- Wassmann, R., Neue, H.H., Ladha, J. K. and Aulakh, M.S. (2004) Greenhouse gas emissions from rice-wheat cropping systems in Asia. Environment, Development and Sustainability, 6, 65–90.
- Wassmann R, Jagadish, S.V.K., Heuer, S., Ismail, A., Redona, E., Serraj, R., Singh, R.K., Howell, G., Pathak, H., Sumfleth, K. And Donald, L.S. (2009a) Climate change affecting rice production: the physiological and agronomic basis for possible adaptation strategies. Adv. Agron. 101, 59-122.
- Wassmann R, Jagadish, S.V.K., Sumfleth, K., Pathak, H., Howell, G., Ismail, A., Serraj, R., Redona, E., Singh, R.K.,and Heuer, S. (2009b) Regional vulnerability of climate change impacts on Asian rice production and Agronomy. Adv Agron 102, 91-133.
- Wassmann R, Jagadish, S.V.K., Sumfleth, K., Hosen,Y. and Sander, B.O. (2010) Rice production and global climate change: scope for adaptation and mitigation activities. In Advanced technolo- gies for coping with climate change ‘no regret’ options for adaptation and mitigation and their potential uptake. Ed. R. Wassmann. IRRI Limited Proceedings No. 16. Los Baños (Philippines). pp 67-76.
- Xu, K.N., Deb, R, and Mackill, D.J. ( 2004) A microsatellite marker and a codominant PCR- based marker for marker-assisted selection of sub- mergence tolerance in rice. Crop Sci. 44, 248–53. I Ye, X., Al-Babili, S., Klöti, A., Zhang, J., Lucca, P., Beyer, P. and Potrykus, I. (2000) Engineering the provitamin A (beta-carotene) biosynthetic pathway into (carotenoid-free) rice endosperm. Science 287, 303-305.
- Ziska, L. H., and Bunce, J. A. (1993). The influ- ence of elevated CO2 and temperature on seed germination and emergence from the soil. Field Crops Res. 34, 147–157.
- Ziska, L.H., Namuco, O., Moya, T. and Quilang, J. (1997) Growth and yield response of field- grown rice to increasing carbon dioxide and air temperature. Agronomy Journal 89, 45-53.
- Zwart, S.J. and Bastiaanssen, W.G.M. (2004) Review of measured crop water productivity val- ues for irrigated wheat, rice, cotton and maize. Agr. Water Management, 69, 115-133.
Figures
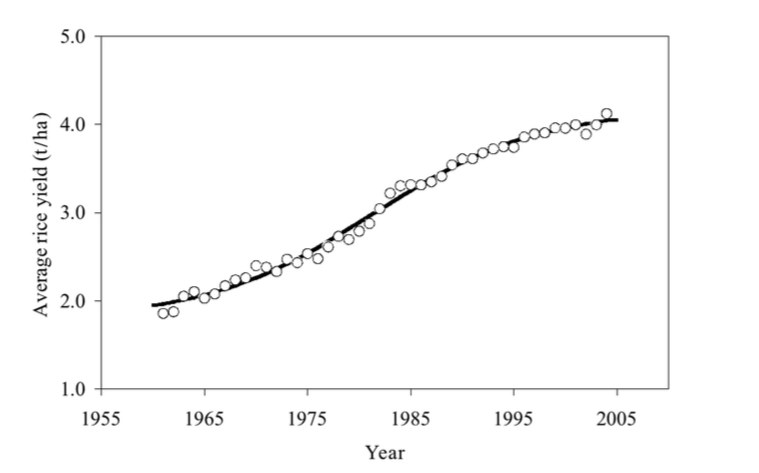
Figure 1: The average yield of paddy rice for Asia between 1961 and 2004. The curve shown is y = 1.78+2.40/(1+exp(-(x-1981)/8.12)) with r2 = 0.99 (data from D. Dawe, personal communication). The curve gives a lower asymptotic yield value of about 1.8 t/ha and an upper asymptotic yield value of about 4.2 t/ha.
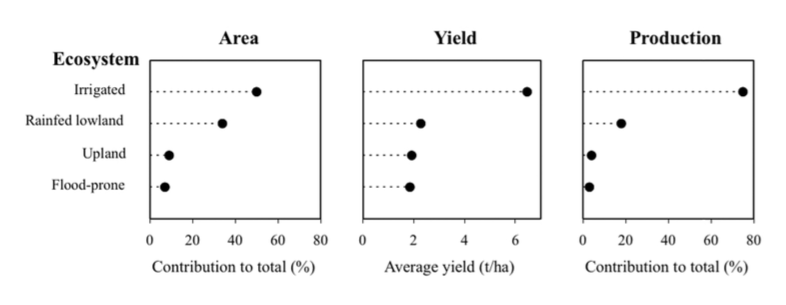
Figure 2: Contributions of the four rice ecosystems to world rice pro- duction. The percentage area and production are from the Rice Almanac (2002). The total area of rice harvested is 157 million hectares and the total production is 677 million tonnes (average of the three years 2007–2009; FAOSTAT 2011) which gives an average yield overall of 4.31 t/ha.
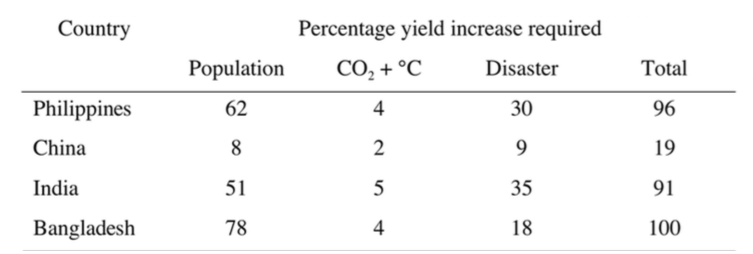
Table 1: Modelled percentage increases in rice yields required by 2050 relative to 2004 resulting from population increases, the combination of increases in temperature and CO2. The increase in yield to allow for disasters (extreme weather events) in four Asian countries is calculat- ed from the data for rice production over the past 40 years from the FAOSTAT database (2005) (after Sheehy et al. 2007a).

Table 2: The mineral element content (kg.ha-1) of a rice crop (grain and straw) yielding grain at 12 t.ha-1 (14% moisture content) with a harvest index of 0.5 (after Sheehy et al. 2001a). Latshaw and Miller (1924) showed that carbon, oxygen and hydrogen made up about 95% of the dry weight of corn. The carbon content of rice plants is approximately 40%; rice straw = 38 % (Jimenez and Ladh, IRRI 2000) and mineral elements comprise about 8%.
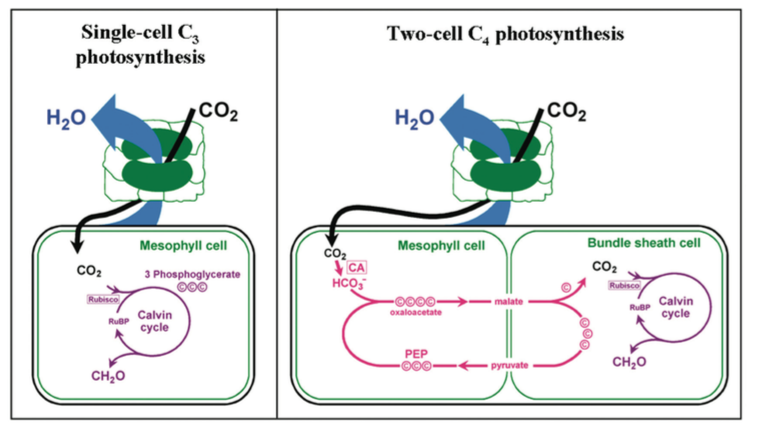
Figure 3: Diagrams of C3 and C4 photosynthesis to summarize the bio- chemistry and show the two types of cell in C4 photosynthesis. The mechanism in the C4 mesophyll cell acts as a pump, supercharging the basic mechanism of carbon assimilation which is restricted to the bun- dle sheath cell. The pump is always operating, like a supercharger, so there is a permanent running cost in metabolic energy which is easy to afford in hot, sunny environments where C4 plants thrive. Rubisco is ribulose 1,5-bisphosphate carboxylase–oxygenase; CA is the enzyme carbonic anhydrase; RuBP is ribulose 1,5-bisphosphate; PEP is phos- phoenolpyruvate; the Calvin cycle is a set of reactions that regenerates the acceptor molecule, RuBP, and adds one unit of fixed carbon in the form of carbohydrate (CH2O) on each turn.
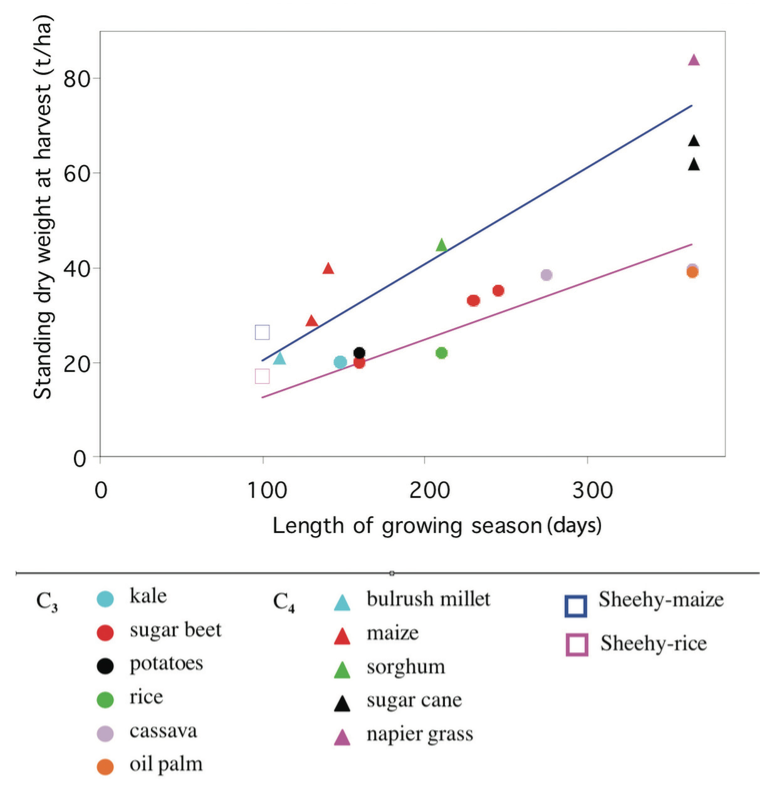
Figure 4: The final dry weights of C4 crops (y = 0·20 x, r2 = 0.85, blue line) and C3 crops (y = 0·12 x, r2 = 0.75, red line) are related to the length of the growing season but with different slopes, i.e. dry weights of C4 crops are 1.7 times those of C3 crops (data from Monteith, 1978). Recent results from maize and rice (Sheehy et al., 2007a) have been added to the original set of points on the graph.
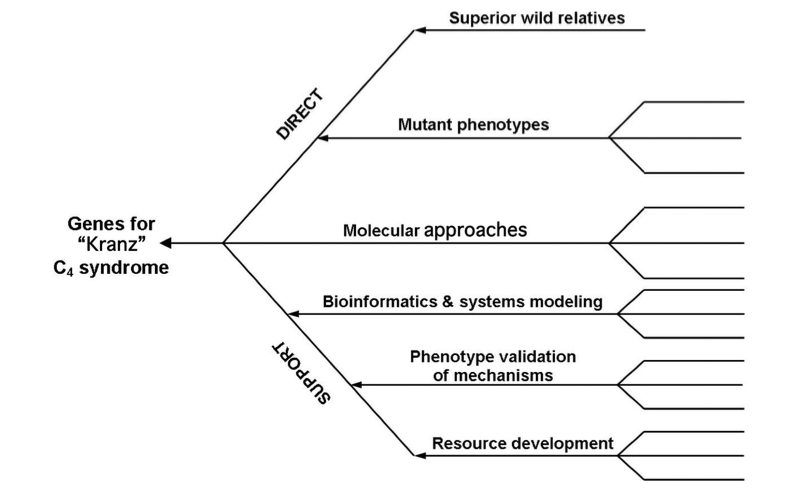
Figure 5: The objectives of the C4 project are shown in two groups: those involving gene discovery (direct) and those concerned with tech- nical developments underpinning the direct approaches (support).
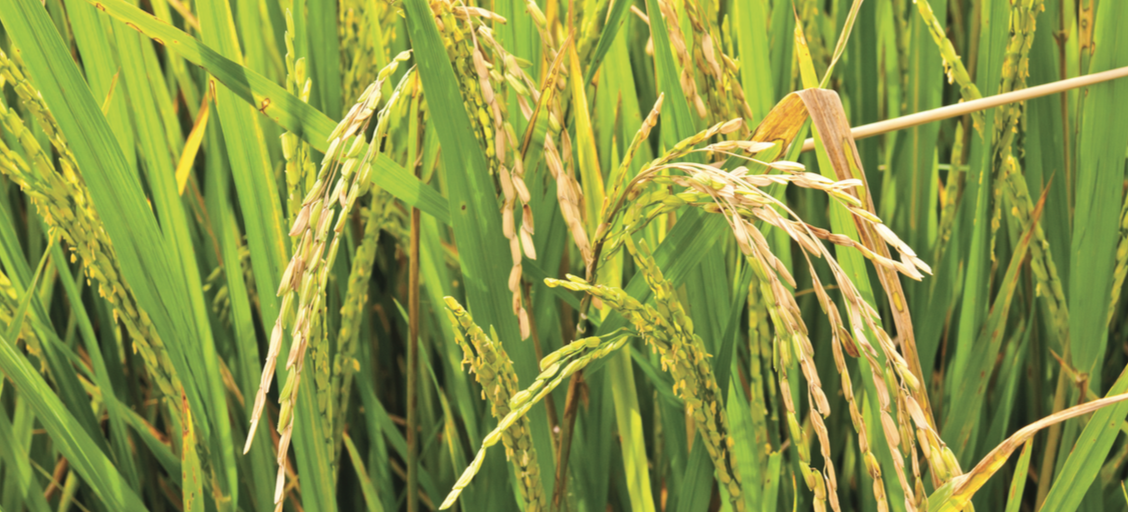
Growing rice plants
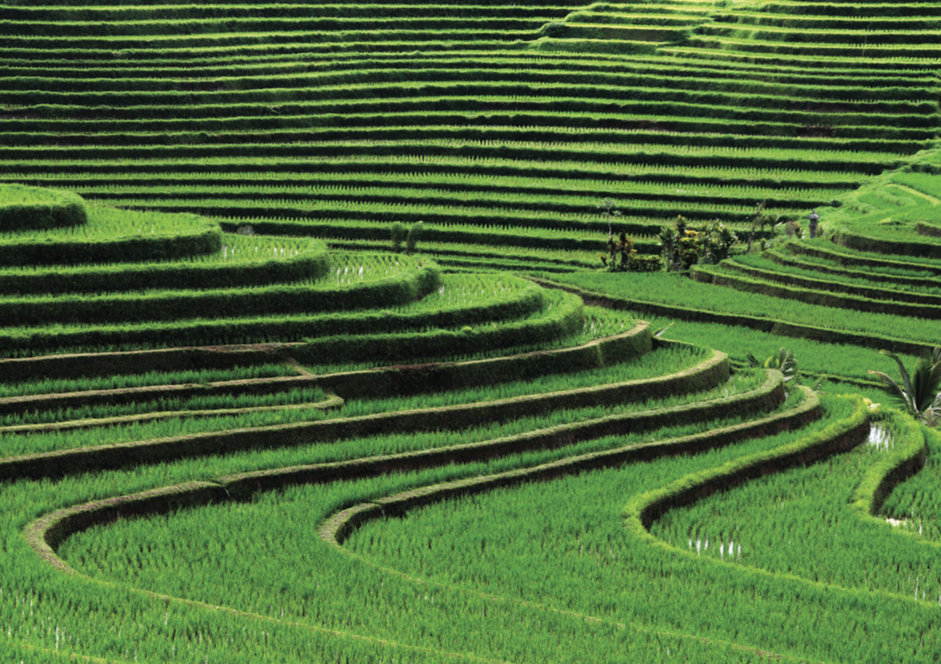
Terrace rice fields, Bali, Indonesia