The supply of fish oil to aquaculture: a role for transgenic oilseed crops?
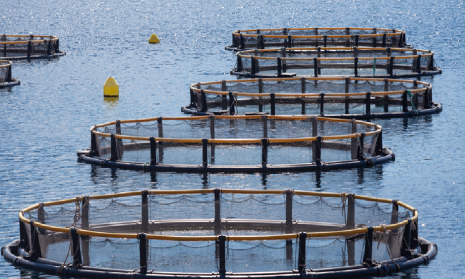
Summary
The importance of an alternative and sustainable supply of omega-3 long chain polyunsaturated fatty acids (LC omega-3) has long been established.
As these biologically active fatty acids have a role in nutrition and health, there is an ever increasing demand for oils containing LC omega-3 e.g. eicosapentaenoic (EPA) and docosahexaenoic acid (DHA). These fatty acids are produced by micoroganisms and enter our diet through the consumption of fish.
However, in order that the nutritional requirements of fish in aquaculture are met and sufficient levels are deposited to meet the requirements of human consumers, EPA and DHA must be supplied in excess.
Given the importance of the aquaculture industry in delivering healthy foodstuff, the question of how to resource the supply of LC omega-3 then arises; traditional sources of EPA and DHA (fish oil) are challenged, whilst vegetable oils do not contain EPA or DHA.
Therefore research efforts have focused on the successful reconstitution of LC omega-3 biosynthesis in oilseed crops. The production of EPA and DHA in the seed oil of agricultural crops has the capacity to deliver large volumes of these fatty acids.
The expression of optimised combinations of the genes required to produce these fatty acids in the seed of the crop Camelina sativa has been achieved and the utility of this approach demonstrated.
This represents a significant breakthrough – the provision of an effective alternative to the use of omega-3 fish oil by the global aquaculture industry.
Key words
Aquaculture; fish oil; nutrition; metabolism; sustainability; GM plants; Omega-3; plant biotechnology
Abbreviations
CVD, cardiovascular disease; DHA, docosahexaenoic acid (22:6n-3); EFA, essential fatty acid; EPA, eicosapentaenoic acid (20:5n-3); FM, fishmeal; FO, fish oil; GM, genetically modified; LC-PUFA, long-chain polyunsaturated fatty acids (≥C20 ≥ 3 double bonds); LC omega-3, LC-PUFA predominantly EPA and DHA; MT, metric million tonnes; TAG, triacylglycerol; VO, vegetable oil.
Introduction
In 2010, fish accounted for around 17% of the global population’s intake of animal protein and almost 7% of all protein consumed (1).
As well as being an important dietary sources of protein, minerals and vitamins (2), fish and seafood are also the major sources of long chain (LC) omega-3 polyunsaturated fatty acids, particularly eicosapentaenoic and docosahexaenoic acids (3), that have well-known beneficial effects in human health, including cardiovascular and inflammatory diseases, and important roles in neural development (4,5).
However, global marine fisheries are stagnating and over 60% of fish stocks require rebuilding (6) so that an increasing proportion of fish are farmed, reaching almost 50% in 2012 (1).
Paradoxically, feeds for many farmed fish species are dependent on fishmeal and fish oil, themselves derived from marine fisheries, for the supply of LC omega-3 (7).
Reliance on finite marine resources was an unsustainable practice (8) and the continued growth of aquaculture has been dependent upon the development of more sustainable feeds with alternative ingredients, generally derived from terrestrial agriculture, that lack LC omega-3 (9).
This has important consequences for the supply of these nutrients to human consumers and so there has been a global drive to find alternative supplies of LC omega-3 fatty acids for aquaculture (10).
The most promising and viable option for entirely new sources of LC omega-3 are transgenic oilseed crops.
2. Long chain omega-3 and human health
It has long been appreciated that dietary LC omega-3 can have important, generally beneficial effects on human health (11).
The strongest evidence has been found in relation to heart and cardiovascular disease (CVD) (12); today a large number of national health agencies and government bodies recognize the importance of increasing dietary intake of EPA and DHA to promote cardiac health and decrease the risk of CVD (12,13).
Recently, the current advice and guidelines worldwide were comprehensively reviewed (14) and recommendations of over 50 organisations were compiled by the Global Organisation for EPA and DHA (15).
Most recommendations suggest between 250 and 500 mg/d of EPA and DHA for reducing CVD risk or 1 g/d for secondary prevention in existing CVD patients, with a dietary strategy for achieving 500 mg/d being to consume two fish meals (200 – 250g) per week with at least one of oily fish (14,16,17) (Table 1).
In addition to cardiac health and CVD, dietary LC omega-3 can be beneficial in several other pathologies with the most robust evidence for inflammatory diseases obtained in rheumatoid arthritis, in which the dose required to gain benefit is set higher than for CVD (around 3g per day (18)).
There is also increasing evidence for beneficial effects of dietary LC omega-3 in Inflammatory Bowel Diseases (IBD) such as Crohn’s disease and ulcerative colitis (19).
The effects of dietary LC omega-3 on cancers has been more controversial. Epidemiological studies indicated that, in general, dietary LC-omega-3 may decrease risk of colo-rectal, breast and prostate cancers (20) and some studies suggest beneficial effects in chemotherapy (21).
However, one research group suggested that LC-omega-3 may be associated with increased risk of prostate cancer (22,23); although latterly a second systematic review and meta-analyses of all studies concluded that the results did not support an association between LC omega-3 and prostate cancer (24).
With regard to development, there is robust evidence that decreased DHA status can lead to cognitive and visual impairment and that DHA supplements have positive beneficial outcomes in pre-term infants (25).
There have also been several reports of potential beneficial effects of dietary DHA supplementation in a number of psychological/ behavioural/ psychiatric disorders including attention deficit hyperactivity disorder and depression, although there are insufficient studies and data to draw firm conclusions (26).
However, it is becoming generally recognised that LC omega-3 are potential key nutrients to prevent various pathological conditions associated with the normal aging process (27), which has prompted research into the effects of LC omega-3 on dementia, including Alzheimer’s disease and other age-related cognitive impairments (28).
In general, DHA supplementation trials in patients with some pre-diagnosed cognitive impairment indicated that this appeared to slow progression of Alzheimer’s (29).
3. Aquaculture: requirement for LC omega-3
Vertebrates, including fish, cannot synthesise polyunsaturated fatty acids (PUFA) because they lack the enzymes required for their production from monounsaturated fatty acids and so they are essential in the diet.
Terrestrial plants can be rich in medium chain PUFA such as linoleic acid and linolenic acid, but the biologically active fatty acids in fish are the long-chain PUFA, primarily EPA and DHA.
Some freshwater and salmonids species of fish can produce EPA and DHA from LNA, but most marine fish cannot.
In consequence, which PUFA can satisfy the essential fatty acid (EFA) requirements in fish vary with species (30,31). The actual EFA requirement in fish can be described at three levels. The amount of EFA a fish requires to prevent nutritional pathology is low, often around 1% of the diet (30,31).
However, a higher level of EFA may be required to support optimum growth and health, which is similar to the situation in humans where few people suffer from EFA deficiency but, rather, LC omega-3 is required for optimal development and health.
The final EFA requirement level in fish is that to maintain nutritional quality based on LC omega-3 content of the flesh (9). As such, this is not a requirement of the fish, but rather that of human consumers (32).
To satisfy this level, EPA and DHA need to be supplied to the fish well in excess of the requirements for optimal health and growth so that they are deposited and stored in the fish.
For example, to produce farmed salmon with the level of EPA and DHA required to supply the weekly human requirement of 3.5g in one 130g portion, it would be necessary for EPA plus DHA to be at 6-7% of diet (33).
This level of supplementation would make fish-farming commercially uneconomical.
4. Fish Oil
In 2013 around 75% of total global fish oil supply was used in aquaculture, with 83% of that consumed by salmonids (60%) and marine fish (23%), and a further 21% used for direct human consumption (34) (Figs.1A &B).
Despite continued growth of aquaculture (1), the use of fish oil for feeds has been relatively stable over the last decade with, on average, around 0.8 million metric tonnes (Mt) being used (34) (Fig.1C).
The primary constraint with fish oil is that it is a finite resource with production limited through strict regulation of fishing and catch quotas (35,36).
Although production of fish oil has declined in recent years, largely due to regulation and quotas in South America (37), this has been partially offset by increased use of seafood and aquaculture by-products including by-catch and trimmings to produce fish meal and, to a lesser extent, fish oil (36).
However, fish oil production averages around 1 million Mt annually and there is little to no prospect of that increasing.
Production of fish oil is also subject to environmental influences and acute phenomena such as El Niño have well-known consequences, considerably reducing supply (38).
Sustainability issues are also key drivers limiting fish oil supplies, and these will have an increasing impact with the many initiatives currently being developed with respect to both national and international standards and certification of marine ingredients, including fish oil (33).
Although increasing adoption and implementation of these standards will further improve sustainability, they will likely have impacts on the availability of fish oil and its use in aquaculture.
A further factor constraining the use of fish oil is the presence of contaminants/undesirables such as persistent organic pollutants (POPS) including dioxins and PCBs (9,39).
With some fish oils, such as those from the Baltic, levels can be higher than permitted for use in animal feeds and these require decontamination before they can be used.
However, this is an issue that has been diminishing to some extent with the increasing replacement of dietary fish oil and as a result levels of these undesirable marine environmental contaminants in feeds are decreasing (40).
To put demand for fish oil (viz. LC omega-3) for aquaculture into perspective, to supply the salmon industry alone would require more than 1 million Mt annually (i.e. greater than the average global supply).
Thus the only way aquaculture has continued to grow has been by increasing substitution with alternative oils (10).
Global oil and fat production was more than 185 million Mt in 2012, with total production of vegetable oils at over 160 million Mt, and animal fats including tallow, lard and butter totalling another 25 million Mt.
Thus, alternative oils are plentiful although they all lack LC omega-3 (Table 2). Terrestrial plants do not produce EPA and DHA and so they are not components of any vegetable oil, whilst animal fats are dominated by saturated and monounsaturated fatty acids with only low levels of PUFA (Table 3).
The consequence of this has been the increasing substitution of fish oil with alternative oils, effectively spreading the available fish oil thinner in the feeds, which has inevitably led to a reduction in LC omega-3 levels in both feeds and farmed fish (10) (Table 4).
However, the issues surrounding the limited supply of LC omega-3 transcend aquaculture.
Based on the most commonly recommended dose for cardiac health (500 mg/day (15)), the demand for LC omega-3 is over 1.25 million Mt whereas global supply is optimistically estimated at just over 0.8 million Mt indicating a shortfall of over 0.4 million Mts (41) (Table 5).
The majority of supply (almost 90%) is from capture fisheries, whether as food fish or via fish oil and meal, with small additional amounts estimated from seafood by-products, unfed aquaculture and algal sources.
The calculations in Table 5 contain assumptions and estimates and so the precise extent of the difference can be the argued, however, that there is a gap between supply and demand is not in question irrespective of how it is calculated (42).
There is a fundamental, global lack of LC omega-3 to supply human needs, whether by direct consumption or via aquaculture.
5. Alternative sources of omega-3 LC-PUFA
There is an urgent need for alternative sources of the LC omega-3, EPA and DHA (41).
As the primary producers of almost all LC omega-3 are marine microalgae and bacteria, the only alternatives to traditional fish oil are other oils sourced from the marine environment including lower trophic levels (zooplankton), mesopelagic fish, by-catch/ by-products and microalgae themselves.
Lower trophic levels
Zooplankton such as krill and calanoid copepods in the southern and northern hemispheres, respectively, are possible options but, although biomass at lower trophic levels is large, there are inherent dangers associated with fishing down the marine food web (43).
Potentially, zooplankton can be good oil sources, but harvesting of krill and copepods poses significant technological challenges and cost (44).
For most species, lack of schooling behaviour makes harvest by traditional trawling technology an expensive economic option (44).
Antarctic krill, which do form schools, are the only species being targeted for commercial harvest, apart from a small scientific quota (~1000 Mt) of the calanoid copepod, Calanus finmachicus (45).
Krill meals, that contain residual oil and therefore some LC omega-3, are currently used in some premium feeds focussed on health benefits and are generally used sparingly.
These krill meals are therefore not being used as primary sources of LC omega-3 and, currently, krill oils are used almost exclusively for the human nutraceutical market.
Although there may be evidence that harvesting krill, and potentially copepods, could be sustainable, there are still significant environmental and ecological concerns (44).
For instance, Antarctic krill are near the base of a food chain that includes whales and penguins, suggesting there could be damage to marine biodiversity (46).
Mesopelagic fish
Mesopelagic fish that inhabit the intermediate pelagic water masses between the euphotic zone at 100m depth and the deep bathypelagic zone at 1000m are available in potentially large quantities (1-6 billion Mt), with lantern fish, myctophids, constituting about 60% of biomass (47).
Different species can contain between 16 and 60% of dry weight (48) as oil and most are potentially good sources of LC omega-3 (44). On the positive side, they are resources that, so far, have not been the subject of commercial exploitation, and they do not compete with existing or potential human feed production.
Negative points include biological (seasonal variation), ecological (mixed fishery difficult to manage), technical (capture methods and on-board processing), and nutritional issues.
By-catch and seafood processing by-products
In almost all fisheries there are non- target catch and/or discarded target catch that, together, make up the by-catch. However, both the precise definition and resultant estimates of by-catch can be controversial (49).
In 2005, the discard rate was estimated at around 7 million Mt/year or 8% of the global catch (50).
By its nature, by-catch is a diffuse resource (51) and this imposes a major limitation to its usefulness as a source of fish oil, although processing of by-products, including oil production, at sea is an increasing trend (52).
Another limitation is that by-catch includes a multitude of species, not necessarily “oily”, which limits the quantity and quality of the oils produced (51).
Seafood industry by-products including viscera, heads, carcasses and trimmings, particularly those produced from pelagic fisheries and aquaculture are another potential source of marine oil although this is largely dictated by species.
Thus, by-products from oily species including salmon, herring and mackerel can be a source of substantial oil whereas by-products from other pelagic (white fish) fisheries have generally low oil contents (53).
Liver from species like cod and halibut have traditionally been used for fish oil production, but production is now relatively small (~ 40,000 Mt) and goes mostly for direct human consumption as vitamin supplements as much as sources of LC omega-3 (54).
Oil is now being actively recovered from aquaculture species waste, particularly salmon farming, with around 20,000 Mt reportedly recovered in Norway (55) and 50,000 Mt in Chile in 2006 (56).
Marine microalgae
Potentially, culture of the main primary producers, marine microalgae, could offer a long-term solution to the sustainable supply of LC omega-3 (57).
Various photosynthetic microalgae are already commonly used in fish hatcheries to supply both EPA (e.g. diatoms) and DHA (e.g. flagellates) in the rearing of larval marine crustacean and fish species (58).
Production usually employs medium- to high- density batch, semi-continuous or continuous culture in relatively small volumes (59).
Up-scaling of production to the volumes required for algal oil and/or algal biomass to supply the amount of LC omega-3 required to replace fish oil in commercial aquafeed production has significant biological and technological challenges (60).
Economic production of LC omega-3 would require algae to demonstrate simultaneous high growth and high oil content with high proportions of EPA and DHA.
These are almost exclusive traits as oil deposition is usually associated with conditions which occur only when growth is limited (e.g. nitrogen limitation) (61).
Technical challenges include efficient capture of light energy in high-density culture with effective temperature control. These issues remain to be solved but there are several research strategies targeting their solution.
These include exploiting culture conditions to direct metabolism towards lipid production, to improve biomass productivity or oil yield by mutagenesis and selective breeding, and to improve strains by genetic modifications to optimize light absorption and increase biosynthesis of EPA and DHA (60).
Therefore, in the future, algal species with favourable biological characteristics may be found and/or developed, and photo-bioreactor technologies may improve considerably enabling economically sustainable production of microalgae rich in EPA and DHA for use in aquafeed.
In contrast, heterotrophic microalgae species including Crypthecodinium and thraustochytrids such as Schizochytrium are already being utilised for the commercial production of DHA using large-scale biofermentor technology (62).
Even so, the high production costs are generally limiting the use of these products to direct human consumption mainly in the form of DHA supplementation of infant formulae (63).
However, the DHA-rich products from heterotrophic microalgae may have niche markets in marine hatcheries, particularly for high-value species.
Production volumes would have to be increased and costs reduced before these products could be viable for wider application in aquaculture.
6. Plants as a source of omega-3 LC-PUFA
It has long been the hope of researchers in the field of plant lipid biotechnology to produce a terrestrial supply of vegetable oil with a composition (EPA/DHA) matching that of fish oil.
However, metabolic engineering on this scale is not trivial. Synthesis of LC omega-3 commonly occurs in marine microalgae via a series of sequential aerobic desaturation and elongation reactions.
These reactions typically follow two routes – the ‘D6 pathway’ (Fig 2a) beginning with the D6-desaturation of the C18 substrate, C2 elongation and D5-desaturation or the much rarer ‘D8 pathway’ where the C18 substrate undergoes a C2 elongation, followed by two rounds of desaturation.
To then generate DHA in either pathway requires a further C2 elongation and desaturation.
Through whichever aerobic route, a minimum of three genes are required to synthesise EPA and five for DHA production.
A further anaerobic route to LC omega-3 has been identified in some bacteria and unicellular marine eukaryotes and functionally characterised; this pathway uses a processive polyketide synthase-like enzymatic system to convert malonyl-CoA to EPA and DHA with the production of no intermediates (64,65).
The activities and genes for each of the biochemical routes to LC omega-3 FA production have been characterised and are available for use by the metabolic engineer to reconstitute EPA/DHA synthesis in a suitable heterologous host (64).
However, success can only be achieved by addressing some significant challenges: (1) the C18 di- or tri-unsaturated substrate must be pre-existing; (2) reconstitution of the pathway in a specific tissue (e.g. seed) requires the co-ordinated and targeted expression of multiple genes and activities; (3) the production of novel LC omega-3 and their correct acyl-exchange between lipid pools depends on the capacity of native endogenous enzymes that are unfamiliar with the fatty acids; and (4) the processes underpinning the biochemical acyl-exchange or flux of fatty acids between lipid species within plant cells is only now starting to be appreciated.
It is entirely possible for biosynthetic intermediates to be channelled into ‘metabolic cul-de-sacs’ rendering them unavailable for further desaturation, elongation or storage in seed oil (65).
Historically, many of the individual genes necessary for LC omega-3 production were individually characterised in plants, indicating that in combination they would be successful. The first demonstration of EPA production in a transgenic plant was published by Qi et al. (66) expressing the algal alternative pathway in the leaves of Arabidopsis thaliana.
The approach used a series of sequential transformations of individual genes and resulted in the vegetative accumulation of EPA, predominantly in phospholipids.
To be of economic value in the context of aquaculture as described above, it is necessary to have an engineered oilseed crop where the target LC omega-3 would be produced and stored in the neutral lipids (triacylglycerol) of seeds at high levels.
The possibility of LC omega-3 biosynthesis targeted to seeds was shown by Abbadi (67) in which the conventional D6 pathway was expressed in transgenic linseed.
Abbadi (67) identified the presence of low levels of EPA in seeds, but concomitant with high levels of C18 D6-intermediates.
The un-wanted intermediates were the result of the poor acyl-exchange of intermediates between the acyl-CoA pool (elongation) and glycerolipid pool (desaturation).
Following these proof-of-concept studies efforts have continued to drive up the accumulation of LC omega-3 in seed oil and reduce the presence of intermediates e.g. D6 desaturation products like linolenic acid.
Such iterative engineering followed two strategies, the first utilised by Petrie and co-workers (CSIRO) made use of Agrobacterium-mediated transient expression of multiple gene combinations in Nicotiana benthamiana (68).
Although testing was undertaken in leaves, the co-expression of the Lec2 transcription factor or master regulator essentially ‘reprogrammed’ the leaf to replicate the lipid composition of a seed; a system that permitted the rapid selection of optimal gene combinations.
The second approach, adopted by Heinz et al. (University of Hamburg) and Napier et al. (Rothamsted Research) was to directly assess gene combinations and their regulatory elements in the seeds of stably transformed plants (namely the oilseed model A. thaliana) (69,70).
In combination with the development lipidomic approaches, it then enabled the evaluation of gene combination efficacy in the seed. Critical to the progress of these efforts was the use of omega-3 desaturases (reducing the accumulation of un-wanted omega-6 fatty acids) and the use of acyl-CoA dependent desaturases (breaking the desaturase/elongase substrate dichotomy) (65).
7. Transition to crops
As described, the majority of the systematic work undertaken to produce a seed oil composition akin to fish oil (i.e. ~20% EPA and DHA in total fatty acids) was undertaken in model plant species i.e. arabidopsis and tobacco.
However, to produce the volumes of LC omega-3 required by aquaculture necessitates the production of EPA and DHA in agricultural oilseed crops.
The opportunities of scale associated with such crops provide the only chance to generate a fish oil substitute in significant quantities.
From the available oilseed crops, screening has identified two as appropriate hosts for the production of LC omega-3; the first canola, a cultivar of rapeseed (Brassica napus L.) and secondly Camelina (Camelina sativa L).
At this time no public data are available to assess the expression of the LC omega-3 trait in canola. However, two laboratories (Rothamsted and CSIRO) have demonstrated the success of Camelina as a host for the accumulation of EPA and DHA in seed oil (71,72).
Camelina is an oilseed crop of the Brassicaceae adapted to temperate growing regions, with a low input requirement, short crop cycle and disease resistance.
In terms of performance, Camelina has an acceptable yield (levels over 3000 kg/ha have been achieved) with an oil content of ~40%.
Of interest to metabolic engineers, Camelina is an excellent platform for the production of tailored oils; it has very low outcrossing levels, and fully developed tools for breeding and molecular trait improvement due to the high sequence identity with Arabidopsis.
Furthermore, genetic transformation is possible using Agrobacterium-mediated floral dip.
However, it is the unique fatty acid composition of Camelina (unusual for a Brassicaceae the polyunsaturated linolenic acid (18:3) and linoleic acid (18:2) – substrates for LC omega-3 synthesis – are the major fatty acids, whilst erucic acid (22:1) is low) that engenders this host to genetic engineering approaches (73).
Although different approaches were taken by Rothamsted and CSIRO, both demonstrated the suitability of Camelina as a host for the production of LC omega-3 in seeds.
Researchers at Rothamsted used knowledge acquired from earlier engineering in Arabidopsis, introducing two optimised constructs into Camelina – one producing EPA alone and the other making both EPA and DHA.
Analysis of the seed fatty acid composition from the stable transgenic lines revealed the average level of EPA alone was 24%, and for EPA and DHA, 11 and 8% respectively (72).
Significantly in both constructs only very low levels of C18 intermediates were recorded.
Overall, the average total level of LC omega-3 was 19% (EPA + DHA) and much higher (25%) in some single seeds (see Fig 2) (72).
The reported work from CSIRO showed significant accumulations of DHA with all the introduced constructs (12% with construct combination GA7 and mod-F), however levels of EPA were low (~3% for mod-F) (71).
The differences in the accumulations of LC omega-3 in Camelina can be attributed to a number of factors e.g. selection of seed-specific promoter, choice of Camelina variety, activity of each enzyme choice, constructs design/orientation, and possibly site of integration.
For example, it is likely that the use of a highly active D5- elongases resulted in the specific difference in EPA accumulation (65).
Moreover, field evaluation of the LC omega-3 trait (EPA + DHA) in Camelina was undertaken at Rothamsted, where the feasibility was demonstrated (by comparison to replicated glasshouse experiments) of producing LC omega-3 in the field without any yield penalty (74).
Thus, the choice of the oilseed crop Camelina as a host for the successful expression of the LC omega-3 trait has been established.
8. Utility of Camelina LC Omega-3 Oil in Aquaculture
The essential question must be; can the novel LC omega-3 oils generated in Camelina be used as a substitute for fish oil?
To address this Betancor et al. (75) recently completed a study in which the EPA-containing Camelina oil described by Ruiz-Lopez et al. (72) was tested as a substitute for fish oil in a salmon feeding trial. In this study, fish oil (control), wild type (WT) and EPA-containing Camelina oil (GM) were fed to juvenile Atlantic salmon within an iso-energetic diet for a period of seven weeks.
At the end of the feeding trial all the fish had doubled in weight and displayed no indicators of ill health; moreover fatty acid analysis indicated the expected accumulation of EPA (as a result of slow EPA conversion, levels of DHA in tissues were also shown to be increased).
Further transcriptomic analysis produced a similar pattern of gene up regulation in fish receiving WT and GM Camelina oil; reflecting the fact that the GM Camelina oil is a modified vegetable oil and therefore contains a different chemical e.g. sterol composition to fish oil (75).
As formulations for aquafeed diets are now composed of blended fatty acids from marine and vegetable sources, the Betancor study successfully demonstrated how LC omega-3 Camelina oil is appropriate for use in aquaculture.
9. Conclusion
As discussed above there is a clear need within aquaculture for an alternative, sustainable supply of LC omega-3.
The potential of engineered oilseed crops to meet this demand have been developed by a number of laboratories over the last fifteen years, but only now, at least with Camelina, has it been possible to demonstrate the viability of oilseed crops as a source of EPA and DHA.
However, it is clear there are a number of hurdles before a Camelina LC omega-3 oil can become a commercial reality.
It must be hoped that, subject to the appropriate regulatory and safety approvals, a forward looking aquafeed industry would be willing to adopt LC omega-3 Camelina oil in fish diets, thereby reducing the pressure on oceanic stocks.
Camelina engineered to produce EPA and DHA, when grown in sufficient volume could make a significant contribution to reducing the demand from capture fisheries (200 000 ha of LC omega-3 Camelina could produce 150 000 Mt of oil or 15% of the global oceanic harvest) (65).
The area required seems large, but it is in fact modest when placed in the context of global vegetable oil production.
For example, such a hypothetical area would be less than 3% of the Canadian oilseed sowing area of a crop like canola (65).
Of course before such numbers can be achieved on farms a number of issues must be resolved; crucially regulatory approval must be sought and obtained, the agronomy and breeding of Camelina must be addressed, oil formulations tested, and the views of consumers understood.
All of these steps require significant effort and resolve, however the demand for LC omega-3 is likely to grow, therefore it is our hope that this experimental terrestrial oilseed source of LC omega-3 can be converted to a commercial reality.
Acknowledgments
Rothamsted Research receives grant-aided support from the Biotechnology and Biological Sciences research Council of the U.K. Some of the work described was supported by BBSRC grant BB/J00166X1.
References
1. FAO, 2014. The state of world fisheries and aquaculture 2014. Food and Agriculture Organization of the United Nations, Rome, 223 pp.
2. Tacon, A.G.J., Metian, M., 2013. Fish matters: Importance of aquatic foods in human nutrition and global food supply. Rev. Fisheries Sci. 21, 22-38.
3. Tur, J.A., Bibiloni, M.M., Sureda, A., Pons, A., 2012. Dietary sources of omega 3 fatty acids: public health risks and benefits. Br. J. Nutr. 107, S23-S52.
4. Campoy, C., Escolano-Margarit, V., Anjos, T., Szajewska, H., Uauy, R., 2012. Omega 3 fatty acids on child growth, visual acuity and neurodevelopment. Br. J. Nutr. 107, S85-S106.
5. Calder, P.C., 2013. n-3 Fatty acids, inflammation and immunity: new mechanisms to explain old actions. Proc. Nutr. Soc. 72, 326-336.
6. Worm, B., Hilborn, R., Baum, J.K., Branch, T.A., Collie, J.S., Costello, C., Fogarty, M.J., Fulton, E.A., Hutchings, J.A., Jennings, S., Jensen, O.P., Lotze, H.K., Mace, P.M., McClanahan, T.R., Minto, C., Palumbi, S.R., Parma, A.M., Ricard, D., Rosenberg, A.A., Watson, R., Zeller, D., 2009. Rebuilding global fisheries. Science 325, 578-585.
7. Tocher, D.R., 2003. Metabolism and functions of lipids and fatty acids in teleost fish. Rev. Fisheries Sci. 11, 107-184.
8. Tacon, A.G.J., Metian, M., 2009. Fishing for aquaculture: Non-food use of small pelagic forage fish – A global perspective. Rev. Fisheries Sci. 17, 305-317.
9. Tocher, D.R., 2009. Issues surrounding fish as a source of omega-3 long-chain polyunsaturated fatty acids. Lipid Technol. 21, 13-16.
10. Turchini, G.M., Ng, W.-K., Tocher, D.R. (Eds), 2011a. Fish Oil Replacement and Alternative Lipid Sources in Aquaculture Feeds. Taylor & Francis, CRC Press, Boca Raton, p.533.
11. Gil, A., Serra-Majem, L., Calder, P. C., Uauy, R., 2012. Systematic reviews of the role of omega-3 fatty acids in the prevention and treatment of disease. Br. J. Nutr. 107, S1-S2.
12. Delgado-Lista, J., Perez-Martinez, P., Lopez-Miranda, J., Perez-Jimenez, F., 2012. Long chain omega-3 fatty acids and cardiovascular disease: a systematic review. Br. J. Nutr. 107, S201-S213.
13. Calder, P.C., Yaqoob, P., 2012. Marine omega-3 fatty acids and coronary heart disease. Curr. Opin. Cardiol. 27, 412-419.
14. Aranceta, J., Pérez-Rodrigo, C., 2012. Recommended dietary reference intakes, nutritional goals and dietary guidelines for fat and fatty acids: a systematic review. Br. J. Nutr. 107, S8-22.
15. Global Organisation for EPA and DHA (GOED) 2014. Global recommendations for EPA and DHA intake (Rev 16 April 2014). http://www.goedomega3.com/healthcare. Accessed November 2014.
16. Gebauer, S.K., Psota, T.L., Harris, W.S., Kris-Etherton, P.M., 2006. n-3 fatty acid dietary recommendations and food sources to achieve essentiality and cardiovascular benefits. Am. J. Clin. Nutr. 83, 1526S-1535S.
17. European Food Safety Authority (EFSA) Panel on Dietetic Products, Nutrition and Allergies (NDA), 2012. Scientific opinion related to the tolerable upper intake level of eicosapentaenoic acid (EPA), docosahexaenoic acid (DHA) and docosapentaenoic acid (DPA). EFSA J. 10(7), 2815.
18. Miles, E.A., Calder, P.C., 2012. Influence of marine n-3 polyunsaturated fatty acids on immune function and a systematic review of their effects on clinical outcomes in rheumatoid arthritis. Br. J. Nutr. 107, S171-S184.
19. Cabré, E., Mañosa, M., Gassulla, M.A., 2012. Omega-3 fatty acids and inflammatory bowel diseases – a systematic review. Br. J. Nutr. 107, S240-S252.
20. Gerber, M., 2012. Omega-3 fatty acids and cancers: a systematic update review of epidemiological studies. Br. J. Nutr. 107, S228-239.
21. Bougnoux, P., Hajjaji, N., Ferrasson, M.N., Giraudeau, B., Couet, C., Le Floch, O., 2009. Improving outcome of chemotherapy of metastatic breast cancer by docosahexaenoic acid: a phase II trial. Br. J. Cancer 101, 1978 – 1985.
22. Brasky, T.M., Darke, A.K., Song, X., Tangen, C.M., Goodman, P.J., Thompson, I.M., Meyskens Jr, F.L., Goodman, G.E., Minasian, L.M., Parnes, H.L., Klein, E.A., Kristal, A.R., 2013. Plasma phospholipid fatty acids and prostate cancer risk in the SELECT trial. J. Natl. Cancer Inst. 105, 1132-1141.
23. Brasky, T.M., Till, C., White, E., Neuhouser, M.L., Song, X., Goodman, P., Thompson, I.M., King, I.B., Albanes, D., Kristal, A.R., 2011. Serum phospholipid fatty acids and prostate cancer risk: results from the prostate cancer prevention trial. Am. J. Epidemiol. 173,1429–1439.
24. Alexander, D.D., Bassett, J.K., Weed, D.L., Barrett, E.C., Watson, H., Harris, W., 2015. Meta-analysis of long-chain omega-3 polyunsaturated fatty acids (LCˆ-3PUFA) and prostate cancer. Nutrition and Cancer 67, 543-554.
25. Carlson, S.E., Werkman, S.H., Rhodes, P.G., Tolley, E.A., 1993. Visual- acuity development in healthy preterm infants: effect of marine-oil supplementation. Am. J. Clin. Nutr. 58, 35 – 42.
26. Ortega, R.M., Rodriguez-Rodriguez, E., Lopez-Sobaler, A.M., 2012. Effects of omega 3 fatty acids supplementation in behavior and non-neurodegenerative neuropsychiatric disorders. Br. J. Nutr. 107, S261-S270.
27. Úbeda, N., Achóna, M., Varela-Moreirasa, G., 2012. Omega 3 fatty acids in the elderly. Br. J. Nutr. 107, S137-S151.
28. Dangour, A.D., Andreeva, V.A., Sydenham, E., Uauy, R., 2012. Omega 3 fatty acids and cognitive health in older people. Br. J. Nutr. 107, S152-S158.
29. Quinn, J.F., Raman, R., Thomas, R.G., Yurko-Mauro, K., Nelson, E.B., Van Dyck, C., Galvin, J.E., Emond, J., Jack, C.R., Weiner, M., Shinto, L., Aisen, P.S., 2010. Docosahexaenoic acid supplementation and cognitive decline in Alzheimer disease: a randomized trial. JAMA 304, 1903-1911.
30. Glencross, B.D., 2009. Exploring the nutritional demand for essential fatty acids by aquaculture species. Rev. Aquacult. 1, 71–124.
31. Tocher, D.R., 2010. Fatty acid requirements in ontogeny of marine and freshwater fish. Aquacult. Res. 41, 717-732.
32. Simopoulos, A.P., 2000. Human requirement for n-3 polyunsaturated fatty acids. Poult. Sci. 79, 961-70.
33. Shepherd, C.J. Monroig, O., Tocher, D.R. (2015) Production of high quality, healthy farmed salmon from a changing raw material base, with special reference to a sustainable Scottish industry. Report for the Scottish Aquaculture Research Forum (SARF Report No. 007; ISBN: 978-1-907266-67-6).
34. IFFO, 2014. The Marine Ingredients Organisation: Fishmeal and Fish Oil Statistical Yearbook 2014. In IFFO [online]. www.iffo.net
35. Shepherd, C.J., Jackson, A.J., 2013. Global fishmeal and fish-oil supply: inputs, outputs and markets. J. Fish Biol. 83, 1046-66.
36. Jackson, A.J, Shepherd, C.J., 2012. The future of fishmeal and fish oil, in: Ryder, J., Ababouch, L., Balaban, M. (Eds), Second International Congress on Seafood Technology on Sustainable, Innovative and Healthy Seafood FAO Fisheries and Aquaculture Proceedings. No. 22. Food and Agriculture Organisation, Rome, 238 pp.
37. FAO, 2012. The state of world fisheries and aquaculture 2012. Food and Agriculture Organization of the United Nations, Rome, 209 pp.
38. FAO/GIEWS, 1997. Food Outlook, Global Information and Early Warning System. No. 10/11/12. October/November/December 1997. Food and Agriculture Organization of the United Nations, Rome.
39. FAO/WHO, 2011. Report of the Joint FAO/WHO Expert Consultation on the Risks and Benefits of Fish Consumption. Food and Agriculture Organization of the United Nations, Rome / World Health Organization, Geneva, 50 pp.
40. Berntssen, M.H.G., Julshamn, K., Lundebye, A.-K., 2010. Chemical contaminants in aquafeed and Atlantic salmon (Salmo salar) following the use of traditional- versus alternative feed ingredients. Chemosphere 78, 637–646.
41. Tocher, D.R., 2015. Omega-3 long-chain polyunsaturated fatty acids and aquaculture in perspective. Aquaculture, http://dx.doi.org/ 10.1016/j.aquaculture.2015.01.010.
42. Naylor, R.L., Hardy, R.W., Bureau, D.P., Chiu, A., Elliott, M., Farrell, A.P., Forster, I., Gatlin, D.M., Goldburg, R.J., Hua, K., Nichols, P.D., 2009. Feeding aquaculture in an era of finite resources. Proc. Natl. Acad. Sci. USA 106, 15103-15110.
43. Pauly, D., Christensen,V., Dalsgaard, J., Froese, R., Torres, F., 1998. Fishing down marine food webs. Science 279, 860-863.
44. Olsen, R.E., Waagbø, R., Melle, W., Ringø, E., Lall, S.P., 2011. Alternative marine resources, in: Turchini, G.M., Ng, W.-K., Tocher, D.R. (Eds.), Fish Oil Replacement and Alternative Lipid Sources in Aquaculture Feeds. Taylor & Francis, CRC Press, Boca Raton, pp. 267-324.
45. Croxall, J.P., Nicol, S., 2004. Management of Southern Ocean fisheries: global forces and future sustainability. Antarctic Sci.16, 569-584.
46. Hill, S.L., Murphy, E.J., Reid, K., Trathan, P.N., Constable, A.J., 2006. Modelling Southern Ocean ecosystems: krill, the food-web, and the impacts of harvesting. Bio. Rev. 81, 581-608.
47. Irigoien, X., Klevjer, T.A., Røstad, A., Martinez, U., Boyra, G., Acuña, J.L., Bode, A., Echevarria, F., Gonzalez-Gordillo, J.I., Hernandez-Leon, S., Agusti, S., Aksnes, D.L., Duarte, C.M., Kaartvedt, S., 2014. Large mesopelagic fishes biomass and trophic efficiency in the open ocean. Nature Commun. 5:3721, DOI: 10.1038/ncomms4271 p.10.
48. El-Mowafi, A., Nanton, D., Berntssen, M., 2010. Evaluation of lantern fish (Benthosema Pterotum) as marine source in fish feeds: nutrient composition and contaminants assessment. Proc. 3rd Global Fisheries Aquacult. Res. Conf. Foreign Agricultural Relations (FAR), Egypt, 29 November - 1 December 2010 pp. 12-23.
49. FAO, 2009. The state of world fisheries and aquaculture 2008. Food and Agriculture Organization of the United Nations, Rome, 196 pp.
50. Kelleher, K., 2005. Discards in the world's marine fisheries: An update. FAO Fisheries Technical Paper 470, Rome, 1.
51. Batista, I., 2007. By-catch, underutilized species and underutilized fish parts as food ingredients, in: Shahidi, F. (Ed.), Maximizing the value of marine by-products. Woodhead Publishing Limited, Cambridge, pp. 171-195.
52. Falch, E., Sandbakk, M., Aursand, M., 2007. On-board handling of marine by-products to prevent microbial spoilage, enzymatic reactions and lipid oxidation, in: Shahidi, F. (Ed.), Maximizing the value of marine by-products. Woodhead Publishing Limited, Cambridge, pp. 47-64.
53. Lekang, O.I., Gutierrez, M.A., 2007. Sources for omega 3 oils (In Norwegian). Rubin, Trondheim, Norway, pp. 106.
54. Hertrampf, J. W., Piedad-Pascual, F., 2000. Handbook of ingredients for aquaculture feeds. Kluwer Academic Publishers. Dordrecht.
55. Rubin, 2009. By-products; www.rubin.no. RUBIN, Trondheim, Norway.
56. Tacon, A.G.J., Metian, M., 2008. Global overview on the use of fish meal and fish oil in industrially compounded aquafeeds: Trends and future prospects. Aquaculture 285, 146-158.
57. Apt, K.E., Behrens, P.W., 1999. Commercial developments in microalgal biotechnology. J. Phycol. 35, 215-226.
58. Muller-Feuga, A., 2004. Microalgae for aquaculture. The current global situation and future trends, in: Richmond, A. (Ed.), Handbook of microagal culture. Blackwell, Oxford, pp. 352-364.
59. Stottrup, J.G., McEvoy, L.A. (Eds.), 2003. Live Feeds in Marine Aquaculture. Blackwell Science, Oxford, 318 pp.
60. Chauton, M.S., Reitan, K.I., Norsker, N.H., Tveterås, R., Kleivdal, H.T., 2015. A techno-economic analysis of industrial production of marine microalgae as a source of EPA and DHA-rich raw material for aquafeed: Research challenges and possibilities. Aquaculture 436, 95-103.
61. Richmond, A. (Ed.), 2008. Handbook of Microalgal Culture: Biotechnology and Applied Phycology. John Wiley and Sons, New York, 584 pp.
62. Raghukumar, S., 2008. Thraustochytrid marine protists: Production of PUFAs and other emerging technologies. Mar. Biotechnol. 10, 631–640.
63. Ward, O.P., Singh, A., 2005. Omega-3/6 fatty acids: Alternative sources of production. Process Biochem. 40, 3627–3652.
64. Kinney, A.J. 2006. Metabolic engineering in plants for human health and nutrition. Curr. Opin. Biotechnol. 17, 130-138.
65. Napier, J.A., Usher, S., Haslam, R.P., Ruiz-Lopez, N, Sayanova, O. 2015. Transgenic plants as a sustainable, terrestrial source of fish oils. Eur. J. Lipid. Sci. Technol. doi 10.1002/ejlt.201400452.
66. Qi, B., Fraser, T., Mugford, S., Dobson, G., Sayanova, O., Butler, J., Napier, J.A., Stobart, A.K., Lazarus, C.M. 2004. Production of very long chain polyunsaturated -3 and -6 fatty acids in plants. Nature Biotechnol 22, 739–45.
67. Abbadi, A., Domergue, F., Bauer, J., Napier, J.A., Welti, R., Zähringer, U., Cirpus, P., Heinz, E. 2004. Biosynthesis of very-long-chain polyunsaturated fatty acids in transgenic oilseeds: constraints on their accumulation. Plant Cell 16, 2734–48.
68. Wood, C.C., Petrie, J.R., Shrestha, P., Mansour, M.P., Nichols, P.D., Green, A.G., Singh, S.P. 2009. A leaf-based assay using interchangeable design principles to rapidly assemble multistep recombinant pathways. Plant Biotech J 7(9), 914-924.
69. Domergue, F., Abbadi, A., Zahringer, U., Moreau, H., Heinz, E. 2005. In vivo characterization of the first acyl-CoA 6-desaturase from a member of the plant kingdom, the microalga Ostreococcus tauri. Biochem J 389, 483–490.
70. Ruiz-Lopez, N., Haslam, R.P., Usher, S.L., Napier, J.A., Sayanova, O. (2013) Reconstitution of EPA and DHA biosynthesis in Arabidopsis: iterative metabolic engineering for the synthesis of n-3 LCPUFAs in transgenic plants. Metab Eng 17:30-41
71. Petrie, J.R., Shrestha, P., Zhou, X.R., Mansour, M.P., Liu, Q., Belide, S., Nichols, P.D., Singh, S.P. (2012) Metabolic engineering plant seeds with fish oil-like levels of DHA. PLOS ONE 7:e49165.
72. Ruiz-Lopez, N., Haslam, R.P., Napier, J.A., Sayanova, O. (2014) Successful high-level accumulation of fish oil omega-3 long-chain polyunsaturated fatty acids in a transgenic oilseed crop. Plant J 77(2):198-208.
73. Vollmann, J., Eynck, C. 2015. Camelina as a sustainable crop: contributions of plant breeding and genetic engineering. Biotechnol. J. 10, 525-535.
74. Usher, S., Haslam, R.P., Ruiz-Lopez, N., Sayanova, O., Napier, J.A. 2015. Field trial evaluation of the accumulation of omega-3 long chain polyunsaturated fatty acids in transgenic Camelina sativa: making fish oil substitutes in plants. Met. Eng. Comm. 2, 93-98.
75. Betancor, M.B., Sprague, M., Usher, S., Sayanova, O., Campbell, P.J., Napier, J.A., Tocher, D.R. 2015. A nutritionally-enhanced oil from transgenic Camelina sativa effectively replaces fish oil as a source of eicosapentaenoic acid for fish. Scientific Reports Article Number 8104:doi 10.1038/srep08104.
Download pdf
Figures
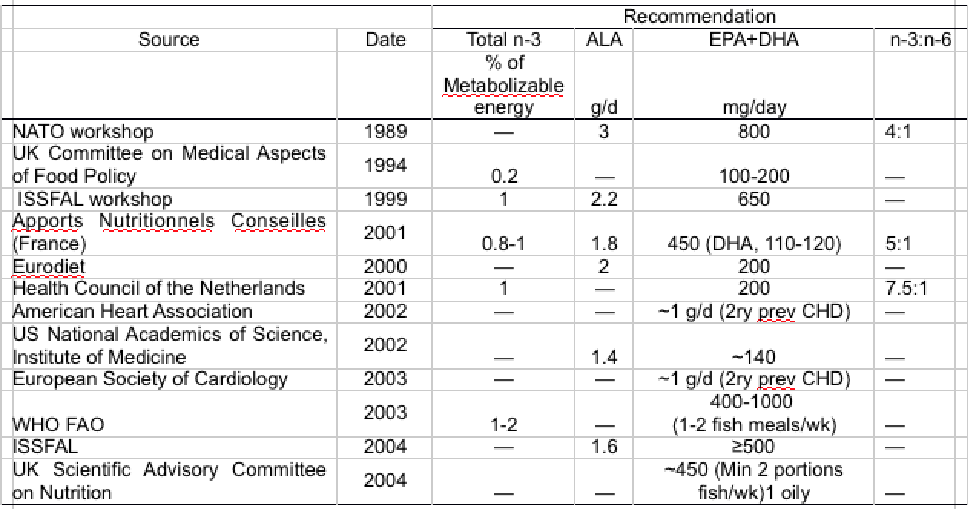
Table 1 International recommendations for dietary long-chain n-3 LC-PUFA consumption in humans. ALA, linolenic acid; DHA, docosahexaenoic acid; EPA, eicosapentaenoic acid; FAO, Food and Agriculture Organization; ISSFAL, International Society for the Study of Fatty Acids and Lipids; NATO, North Atlantic Treaty Organization; WHO, World Health Organization.
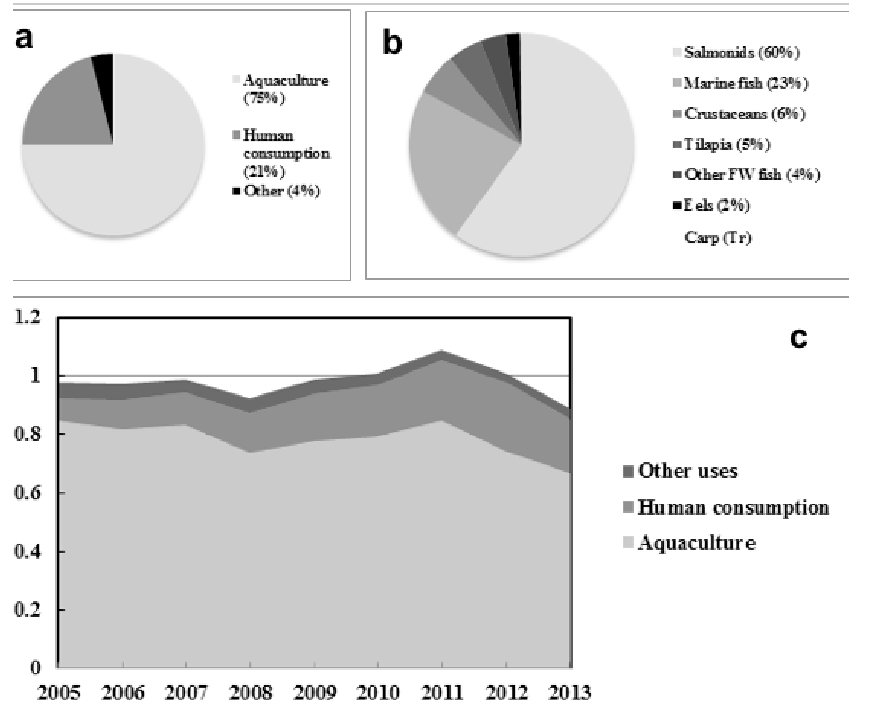
Figure 1. Global Consumption and supply of fish oil. In 2013 around 75% of total global fish oil supply was used in aquaculture, 83% of that consumed by salmonids (60%) and marine fish (23%), and 21% went human consumption (a & b). The use of fish oil for feeds has been relatively stable over the last decade on average around 0.8 million metric tonnes (34) (c).
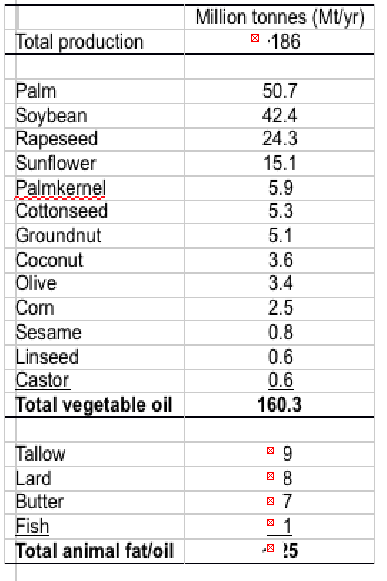
Table 2. World oil and fat production in 2012 (a). a http://lipidlibrary.aocs.org/market/ ofo6-07.htm (Updated 03/2013; accessed 10/2014)
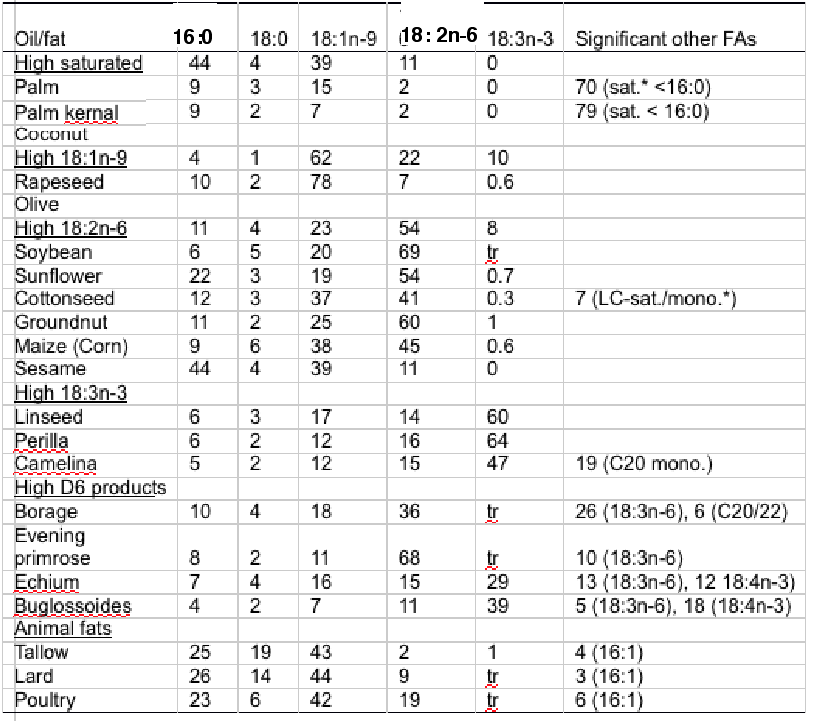
Table 3. Fatty acid (FA) composition (Mol%) of major vegetable oils and animal fats. *Sat.,saturated FA; Mono., monounsaturated FA
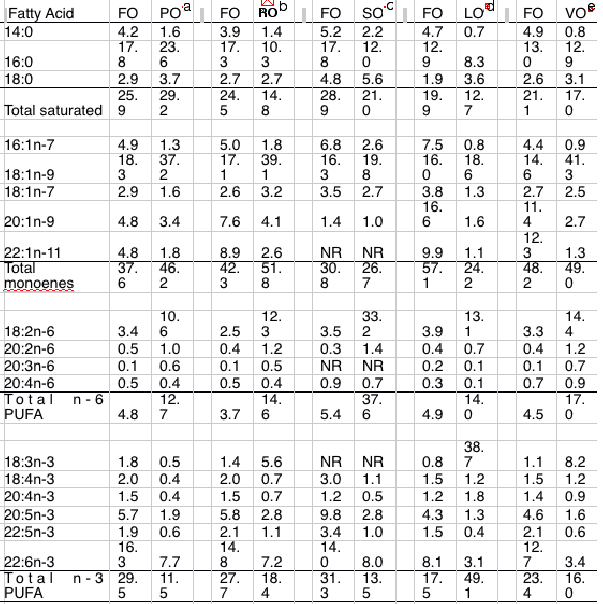
Table 4 Effect of complete or partial replacement of dietary fish oils by vegetable oils on fatty acid compositions (percentage of weight of total lipid) of flesh of Atlantic salmon. FO, fish oil; LO, Linseed oil; PO, palm oil; RO, rapeseed (Canola) oil; SO, sunflower oil; VO, vegetable oil blend.Details of fish initial weight, extent of dietry replacement and length of feeding trial are provided: a Initial wt. 55 g / 100 % replacement / 30 weeks, b Initial wt. 80 g / 100 % replacement / 17 weeks, c Initial wt. 22 g / 100 % replacement / 9 weeks, d Initial wt. 127 g / 100 % replacement / 40 weeks), e Initial wt. 0.16g / 100 % replacement, RO/PO/LO [3.7:2:1] / 22 months.
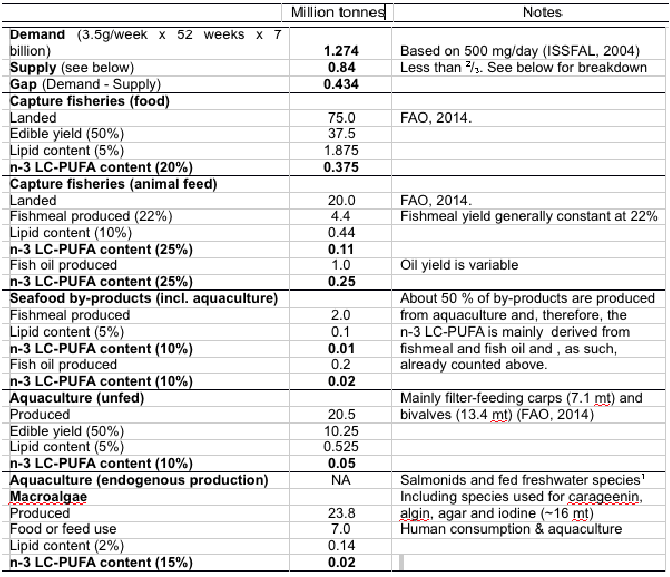
Table 5. Potential annual demand for n-3 LC-PUFA based on recommended intake for reduction of risk of cardio-vascular disease compared with global annual supply from major sources. Production figures are from 2012 (FAO, 20141). All lipid and n-3 LC-PUFA contents/yields are estimated averages over the wide range of species in each category. 1Salmonids include salmon and trout spp. Freshwater species includes eels, tilapia and other freshwater species that still utilise some fishmeal and fish oil (13% of total fish oil used in aquaculture) in feeds. NA, not available. Although there will be some endogenous production of n-3 LC-PUFA inversely related to fishmeal and oil contents of feeds, this cannot be accurately estimated, but will likely be minor based on volume of fish oil consumed by these sectors (75% of all fish oil used in aquaculture).
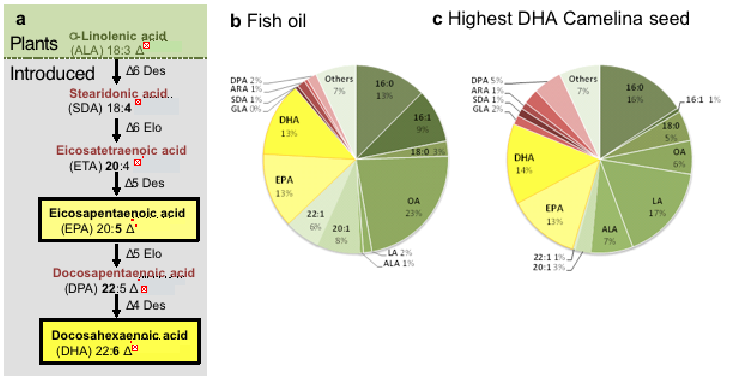
Figure 2. The biosynthesis pathway of LC omega-3 in Camelina. (a) Schematic representation of the enzymatic steps involved in the synthesis of LC omega-3 fatty acids; total fatty acid composition of generic fish oil (b) and an individual seed of GM Camelina (72).